For patients with intractable epilepsy, the role of intracranial surgery has been progressively recognized as a well-tolerated and clearly beneficial treatment option.1–3 Improvements in quality of life are now frequently documented alongside the success rates with surgical approaches, and early surgical consideration for medical failures has gained acceptance as a routine part of the comprehensive approach to refractory seizure management. Although some patients can proceed to an operative intervention based on clinical presentation and noninvasive tests,3,4 others require more investigation before surgical resection can be considered. This latter group may have insufficient scalp electroencephalography (EEG) documentation of their seizure disorder or an unknown correlation between seizures with a suspected lesion or substrate; they also may lack localization of an ictal onset that is amenable to surgical resection. In other cases, surgical resection for seizure control may pose significant risk if adjacent regions harbor the eloquent cortex. When language, motor, or sensory function may be involved, intracranial electrodes can be used for functional mapping.
In these more challenging cases, subdural strips and grid electrodes have become more important and increasingly useful.5,6 Intracranial EEG and stimulation can provide the final necessary information that permits consideration of a surgical approach. This chapter will discuss factors influencing the strategies chosen for subdural electrode use when evaluating a patient with partial epilepsy.
EEG provides a noninvasive physiological examination of the brain that allows characterization of neuronal discharge patterns. It provides a graphic representation of frequency and amplitude, and also demonstrates the temporal relationship and spatial distribution of electrical activity from the cerebral cortex.7 EEG identifies both normal and pathologic states, with the latter role being essential in the diagnosis and treatment of seizure disorders.8,9 However, the role of a standard EEG is limited in that the typical events of interest (seizures) do not reliably occur while the test is performed.10,11 To address this dilemma, long-term, continuous, noninvasive monitoring can be performed in an epilepsy monitoring unit (EMU). The EMU also provides a safe environment where supervision in an inpatient environment minimizes the risk when medications are tapered to help capture a seizure.
Despite the ability of an EMU to greatly improve the likelihood of capturing an event, there are still significant limitations with scalp EEG.12 The use of scalp contacts requires that the electrical activity of the brain must be detectable through several layers of tissue, each of which causes various impediments to the process of recording an EEG. When using superficial electrodes, the scalp reduces the amplitude of EEG signals. Even trying to abrade the scalp to improve the conductivity does not provide a significant improvement in the EEG.13 Studies of bone indicate that it is a poor conductor of electrical energy.14,15 The skull, dura, and cerebrospinal fluid (CSF) have been demonstrated to attenuate the signal strength of the brain’s activity, affecting amplitude,16 and with attenuation, the ability to discern true signal from ambient noise is reduced. Without the effects of the skull and scalp, subdural electrode amplitudes are more than an order of magnitude greater than signals reaching the scalp. Also, although long-term scalp electrodes require attention and the application of electrolyte, subdural electrodes have reliably low impedances that are maintained during long-term monitoring.
Artifacts, or extraneous noise relative to true cortical electrical activity, are another limitation of scalp EEG, even in an EMU. So-called internal artifacts are related to concomitant patient physiology, such as electrocardiogram activity, eye movement, swallowing, and other voluntary or involuntary muscle movement. External artifact may arise from any source of electrical interference, including 50 or 60 cycle noise from standard alternating current or the use of adjacent electrical equipment. This latter effect can be more prevalent in the inpatient setting of the EMU, given the amount of technology in the hospital environment.17 Additional artifact results from irregularities in the skull, such as after craniotomy, and produces a “breach rhythm.”18 Subdural electrodes provide a stronger signal for recording (better signal-to-noise ratio) and are not subject to most of the other artifacts noted above.
Upon passing through the calvarium and scalp, electrical currents experience some diffusion, or “smearing,” and can appear over a greater distribution than the focal nature of their onset.19,20 This limits the accurate localization of an epileptic focus. The frequency of scalp EEG recordings is also distinct from what is obtained from subdural electrodes. Scalp EEG demonstrates only a portion of the high frequencies seen on subdural recordings. However, this is not felt to be due to the skull and scalp as much as the result of summation of polyphasic cortical activity.21
Another aspect that limits scalp EEG is the irregular geometric relationships between the scalp (determined by the skull) and much of the brain’s cortical surfaces. Although the scalp approximates the surface of a sphere, the underlying cortex has a much more complex shape with infolded gyri and deep clefts at the sylvian and interhemispheric fissures. Because scalp EEG is most sensitive to radial (perpendicular) oriented discharges or dipoles,22 electrical activity coming from cortical discharges not in this orientation may be poorly discerned. Another problem related to spatial relationships is the longer distance between the basal regions of the brain and external electrodes. This distance attenuates the electrical signal such that it is poorly detected at the scalp.23 With regard to all issues mentioned, placement of subdural electrodes overcomes many of these obstacles.
The greater sensitivity when EEG contacts are on the brain versus the scalp is well illustrated by the work of Tao and colleagues.24 They recorded simultaneous scalp EEG while monitoring intracranial EEG during evaluation for temporal lobe epilepsy surgery. The group compared cerebral discharges with and without scalp EEG activity and estimated the volume of brain involved by the number of subdural contacts having concurrent depolarization. Monitoring interictal spikes, they found that the source area had to reach a critical size before reliably producing recordable scalp potentials: 90% of cortical spikes with a source area >10 cm2 produced scalp EEG spikes, whereas only 10% of cortical spikes having <10 cm2 of source area produced scalp potentials. They also found a minimal threshold where source areas <6 cm produced no scalp potentials. This last finding is consistent with the much earlier work of Cooper et al.25
The limitations of sensitivity and spatial resolution of scalp EEG diminish its utility in trying to localize the focus of partial events when considering surgical options. In distinction, subdural electrodes can provide the precise localization necessary for both complete resection and preservation of the functional cortex because each electrode is quite specific for the underlying cortex.
Finally, noninvasive EEG may not demonstrate any pathology in certain abnormal states, particularly partial seizures. Seizure types that have been shown to occur in the absence of EEG abnormalities include seizures of extratemporal origin (particularly frontal lobe), simple partial seizures, and complex partial seizures that do not generalize.26,27 Spencer et al. estimated that noninvasive EEG is unable to sufficiently identify the epileptogenic zone in ~25% of patients with medically uncontrolled epilepsy.28
This first case study demonstrates how subdural electrodes can obtain a much cleaner EEG relative to scalp recordings. The study also demonstrates two limitations of subdural recordings. Even with invasive monitoring, precise localization of a seizure onset may still be difficult (the intracranial EEG in Videos 32-1a and 32-1b revealed only regional suppression). Furthermore, subdural electrode data may be limited if the onset appears at the edge of the grid (edge effect). Moving or adding grids to mitigate these issues is discussed in the section Electrode Selection and Placement.
Case Study 1
Scalp EEG vs. subdural EEG
A 19-year-old right-handed male patient was referred for intractable epilepsy beginning at the age of 15. His spells began after a minor closed head injury with questionable loss of consciousness. His seizures would begin with right arm automatism, during which he would rub his right face. At night he would begin with right arm twitching and also get out of bed and crawl in counterclockwise circles, which his parents have likened to “chasing his tail.” He was admitted for a long-term (5-day) video-EEG monitoring (VEM) session, resulting in the recording shown in Videos 32-1a and 32-1b. In the segment shown, the most obvious issue was the significant amount of muscle/movement artifact that obscures most of the recording. The patient had negative magnetic resonance imaging (MRI) and nuclear medicine studies, including SISCOM (subtraction ictal SPECT [single-photon emission computed tomography] co-registered to MRI). Implantation of strips and grids was recommended for localization and evaluation for other therapy. The patient was subsequently admitted for phase 2 studies with subdural electrodes (Figure 32-1). Video-EEG in the EMU was performed after implantation, with a representative segment shown in Videos 32-1a and 32-1b. One now clearly sees the greater clarity of the recording that is not longer obscured by artifact.
Figure 32-1.
(A) Anteroposterior and (B) lateral skull films for Case Study 1. Right frontal subdural strips (arrows), a dual-sided interhemispheric grid (triangle), and left frontal convexity (a), subfrontal (b), and temporal (c) grids are shown.

Noninvasive EEG, while providing the majority of information for the diagnosis and characterization of epilepsy, may prove insufficient for more complex cases. Invasive EEG can overcome many of the technical limitations of scalp recordings, and when considering surgical resection in complex cases, intracranial EEG becomes a significant adjunct if not mandatory part of the evaluation.
Three-dimensional neuroimaging techniques have advanced greatly from initial anatomical detail provided by computed tomography (CT) and MRI.29 The detailed examination of intracranial anatomy is now part of the routine evaluation for patients with epilepsy,30,31 and the appearance on MRI of typical lesions associated with epilepsy have been well defined.32 Despite the strides in neuroimaging, many patients with epilepsy will still have negative imaging studies.33
Imaging of the normal and abnormal physiological epiphenomena in the brain falls within the category of functional imaging. These tests include positron emission tomography (PET), magnetoencephalography (MEG), and SPECT.34 Ictal SPECT has been noted to be helpful as a guide to optimize placement of intracranial electrodes. In successful cases of epilepsy surgery, there appears a high rate (70%) of colocalization between the resected area and the area of maximal increased perfusion identified by ictal SPECT.35 More recently, differential SPECT imaging of ictal activity has been combined with anatomical detail to help localize seizure onset. SISCOM has been shown to have increased utility over SPECT for imaging ictal onset.36 Similarly, MEG data can give localizing information about interictal activity.37 However, while providing additional information, SISCOM or MEG findings alone are not usually sufficient to determine either resectability or the extent of resection, particularly in extratemporal cases. Although noninvasive imaging data may not be conclusive, using functional imaging superimposed on MR anatomy can be helpful to plan subsequent subdural monitoring38 (Figure 32-2). The results of seizure control are much improved when resection is guided by SISCOM with subsequent intracranial monitoring. The results of postsurgical seizure control are 60% excellent when the SISCOM abnormality is resected versus 20% excellent when resection does not include the SISCOM focus.36 PET imaging typically identifies regions of hyper- or hypometabolism. However, the practical use and technical needs of PET limit capturing ictal events reliably, similar to MEG. Interictal PET is commonly used to identify regional hypometabolism, such as in medial temporal sclerosis (MTS). In cases where it correlates well with temporal lobe epilepsy, it may be sufficient to guide resection. Additionally, in identifying extratemporal abnormalities, current PET studies may provide information that could support other results, but by themselves they will not likely identify focal epileptogenic regions or guide resection.
Figure 32-2.
(A–D) Coronal, fluid-attenuated inversion recovery (FLAIR) sequences of a seizure patient with extensive cortical dysplasia (arrows). The anomaly extends from (A) the anterior temporal to (D) the posterior parietal regions. (E) Sagittal and (F) coronal subtraction ictal single-photon emission computed tomography (SPECT) co-registered to magnetic resonance imaging (SISCOM) views reveal the superficial, focal location of seizure onset (yellow) within the more widespread dysplasia. Such localization can suggest a more directed surgical implant, avoiding a larger craniotomy and using fewer electrodes, both of which can reduce the risk of complications.

Most patients with temporal lobe epilepsy, ipsilateral MTS on MRI, and concordant hypometabolism on PET can move directly to resective surgery without additional studies. However, there is a small population of patients with MTS with an indication for intracranial monitoring. Approximately 10% of patients with MTS have a lack of concordance between the side of hippocampal atrophy on MRI and the EEG abnormality.39,40 Thus, subdural (or depth) monitoring may be able to resolve discordance or “rule in” a temporal lobectomy on the side of radiographic MTS. In concert with this is evidence that one out of seven patients with MTS has another lesion that might be a cause for seizures, such as a vascular malformation, low-grade tumor, or malformation of cortical development.41 Properly identifying the cause of seizures in these dual-pathology patients is important, as the results of removing the extratemporal lesion alone are not associated with a high rate of seizure freedom.42 Technologies that increase the sensitivity and specificity of MRI and other modalities will likely advance with time to better detect epileptogenic lesions.43 However, there will continue to be a role for subdural monitoring whenever there is suspicion of a cortical focus and ambiguity between EEG and the anatomical findings or when there are dual or multiple pathologies.
When there is concordance between EEG, anatomical, and functional imaging data, there is likely no need for intracranial monitoring.44 Yet with disparities in data, the role of both anatomical and functional imaging can be employed to guide intracranial electrode implantation. With an ability to confine implantation to a specific region of interest, the extent of implant surgery may be reduced yet would still cover the most suspected areas. Because the risk of complications has been shown to increase 40% with every additional 20 subdural electrodes45 and >80% with bilateral implantation, the modalities of imaging and subdural implantation become complementary to minimize patient risk and increase the yield for ictal zone determination and successful surgery.
The precursor to implanted subdural electrodes was their use intraoperatively as pioneered by Berger in 1929. These initial investigations were performed with the electrodes placed outside the dura in patients with skull defects.46 Others have also championed the use of epidural electrodes in various formats,47–49 typically because of the ease of insertion and the lower potential for infection. However, the desired result of increased sensitivity and greater spatial resolution with epidural placement is still inferior to subdural electrodes that lie directly in contact with the cortex.50 Penfield was the first to document the use of subdural electrodes with a surgical procedure.51 This was presented around 1940, but it was more than 40 years before frequent reports began to appear in the literature describing the use of subdural grid electrodes.5 Although the use of subdural electrodes has become more common, no standardized approach has been identified. Variability exists in indications, implant techniques or paradigms, and the number and type of electrodes.5 Without agreement on a uniform approach, it becomes difficult to make fair comparisons between institutions or any assessment of methods, and a multitude of preferences exist. However, there are clearly advantages to specific approaches. Monitoring regions of the cortex such as the subtemporal and interhemispheric areas cannot be reached with epidural electrodes, yet they are accessible to subdural electrodes, typically via craniotomy. In frontal lobe epilepsy, medial frontal discharges are not detected with scalp EEG52 and thus are an excellent indication for subdural electrodes. Regardless of any preference or approach, any time scalp recordings cannot confirm correlation between an imaging abnormality and the patient’s seizures, invasive monitoring is indicated. Even in lesional cases, the best surgical outcomes have been found to occur when an MRI visible focus is consistent with an EEG focus and can be completely removed.53
The choice of electrode strips versus grids is based on the location, accessibility, and degree of coverage desired of suspected epileptogenic regions. Subdural strips (a single line of electrodes) have a lower risk than grids by virtue of their smaller size and may be inserted via a burr hole, thus avoiding a full craniotomy. Subdural electrodes are usually manufactured from soft, flexible, thin transparent Silastic sheets that have small metal contacts and wires embedded within them. Although both stainless steel and platinum contacts are available, the MRI compatibility of the latter allows useful postimplant imaging. Some centers make their own strips and grids.5 Commercial providers offer an array of choices in varying dimensions (e.g., Ad-Tech Medical Instrument Corp., Racine, Wisconsin, and PMT/Permark Corp., Chanhassen, Minnesota). A common configuration spaces the 5 mm electrode centers 1 cm apart. The length and shape of the strip/grid are chosen after a review of the noninvasive EEG recordings, imaging studies, and other clinical information, such as seizure semiology and language localization. Various sizes are available; we have found 4 × 5 cm grids to be useful for subfrontal (orbitofrontal) and subtemporal coverage, whereas larger 6 × 8 and 8 × 8 cm grids are useful when covering large areas of the cerebral convexity. Subdural strips have also been used to supplement grids, add regional coverage, or monitor medial temporal lobe regions.54 Dual-sided strips and grids are also available for placement in the sylvian fissure, within schizencephalic abnormalities, and in the interhemispheric fissure. Both strips and grids have electrode leads that exit the Silastic sheet and must be brought through the dura and bone upon closure of the surgical wound. These leads are tunneled under the galea and exit through small sites some distance from the scalp incision. Case Study 2 demonstrates an implant methodology on a nonlesional case.
Case Study 2
Nonlesional, frontal lobe epilepsy
A 23-year-old man began to have events at age 13 characterized as staring spells and being unable to speak, and was diagnosed as having “panic attacks.” His first generalized convulsion changed his diagnosis to a seizure disorder, but he subsequently failed six medications. Furthermore, despite instructions otherwise, he continued to drive. His MRI, PET, and SISCOM studies were all normal. During his EMU admission, 35 events were recorded, with the scalp EEG consistently showing right frontocentral onset. He underwent craniotomy for right frontal grid placement predominantly in the central and orbitofrontal regions, with one strip over the right temporal lobe (Figure 32-3A). His EMU EEG repeatedly showed onset from electrodes 37, 45, and 29 in the large frontal grid (Figure 32-3B). Stimulation at the region of interest had no effect on motor, sensory, or language function, although stimulation at the posterior and inferior margin of the grid did cause positive motor responses. He was taken for surgery, where the grid was removed and the area surrounding the onset zone resected (Figure 32-3C,D,E). He had an uncomplicated recovery and has remained seizure-free. Although the intraoperative images may look dramatic, the true perspective of the focal nature of resections planned with subdural monitoring is demonstrated on the patient’s postoperative MRI (Figure 32-3F).
While the obvious goal during implantation is to cover the area of suspected ictal onset, some invasive monitoring recordings fail to positively identify the seizure onset. In these cases, staged procedures, repositioning, or the use of additional electrodes can provide the necessary information to complete a successful implant procedure.55,56 Additionally, depth and subdural electrodes used either together or sequentially is not mutually exclusive. Case Study 3 illustrates this.
Figure 32-3.
(A) Anteroposterior (left) and lateral (right) skull films showing right frontal convexity (8 × 8) and right orbitofrontal (4 × 5) grids. A subdural strip electrode (1 × 8) can be seen on the lateral view covering the superior temporal region. The red dot identifies electrode 37 (see B–E). (B) Electroencephalography (EEG) from the 8 × 8 contact grid shown in A. Seizure activity begins at contacts 37 (red arrows), 45 (black arrows), and 29 (gray arrows), in that order. These three electrodes were in line next to each other in adjacent rows in the center of the large frontal convexity grid. The EEG lines are colored blue and black to allow visualization of each row of eight electrodes. (Top left) Intraoperative view at the time of explant/resection following monitoring. The view is from the neurosurgical position (upside down), with the patient facing left. The right temporal lobe is at the top, and the subdural strip is seen just beyond a vein in the sylvian fissure. The frontal lobe is at the lower portion of each image and is covered by the large grid. Electrode 20 and the lead of the orbitofrontal grid can be seen on the left side of the photo. (Bottom left) The large 8 × 8 grid has been removed after locations of electrodes 37 and 45 were marked with bipolar coagulation on the pial surface. This is the center of the region to be resected. (Top right) Right frontal lobe following focal cortical resection. The field of resection included a 2 cm margin in each direction outside the three sites of ictal onset except in the posterior and inferior direction, where it was limited to a 1 cm margin. This protected the closest region of the motor cortex identified by preoperative mapping (white triangle). Resection of the cortex allows visualization of the subcortical white matter. (Bottom right) Magnetic resonance imaging (MRI) (postcontrast T1-weighted images) following surgery. Coronal (left) and axial (right) views demonstrate the expected postoperative defect.

There are a few distinct groups of patients with whom common or uniform strategies of implanting electrodes may apply. Examples of populations where this may be seen include clinically suspected temporal lobe epilepsy with very rapid spread/generalization. When this occurs in the presence of bilateral MTS or bilateral temporal hypometabolism,57,58 equivocal PET data, or in nonlesional cases, bilateral temporal subdural strips may be a standard implant routine. Bilateral hippocampal depths may also be chosen for these situations. However, as noted previously, there is no standardized methodology regarding choice of subdural strips, subdural grids, depth electrodes, or some combination thereof. Although various authors have expressed their preferences, the common theme in choosing an implant methodology is that each strategy is tailored to the specific clinical needs of each patient. The basic goals are to define as best as possible the focal onset of a seizure and delineate any regions of eloquent brain (usually language or motor function). In lesional cases where the correlation between the lesion and the seizure are highly suspected by noninvasive data, no implant may be necessary. However when there are disparities, the implant plan would clearly include coverage of the lesion, as well as coverage of whatever region of the brain is suspected by EEG or imaging studies (MRI, PET, MEG, and SISCOM). In some cases confirmation can be achieved by use of several strips placed via burr holes. This is the least invasive manner of using subdural electrodes. However, the caution with subdural electrodes is that placement is done without direct visualization. Although delicate subdural structures such as veins may be encountered while sliding strips into a desired location, the relative safety of subdural strip placement is well documented.59 Because subdural strip placement can be done relatively easily, strips are sometimes employed to determine regional onset and define or “rule in” the possibility of a more extensive subdural grid implantation. Such would be the case when there is rapid spread of EEG and laterality of onset is not clear, when extensive or multiple lesions are present in different lobes of the brain, or when no lesions are present, and a uniform, stereotyped, but nonlateralized onset is noted. Sometimes strips are used as part of a staged surgical plan that guides subsequent implantation of a subdural grid strategy. In these cases, strips can help define laterality and perhaps regionally, while follow-up implantation of grids can define discrete focality. Although extensive grid placement could be an alternative approach in some of these circumstances, a staged methodology may help avoid an extensive craniotomy using a large number of grid electrodes, and the increased risk proportional to the number of grid electrodes has been documented. Difficulties with subdural strips through burr holes include a limited ability to guide placement over focal (vs regional) areas of interest and problems with adhesions between the dura and the cortex. This can occur when there has been prior surgery for tumors or trauma or in cases with a past history of infection. When placement is not critical, the surgeon can attempt to modify the location of a strip by sliding it to one side of an obstacle. However, burr hole insertion of strips is routinely accomplished over the cerebral convexities and has even been performed to place strips covering the medial and basal temporal lobes and in the interhemispheric fissure.
Case Study 3
Staged implant procedure
A 24-year-old, right-handed man presented with a history of having several seizures per month despite multiple medications. The seizures began at the age of 14 with a history of encephalitis. The seizures are typically 1 to 2 minutes of a complex partial type. During VEM, he had five complex partial seizures, four starting in the left temporal region and one nonlocalized onset. The patient also had bilateral interictal discharges, but right greater than left. Neuropsychology testing suggested both dominant and nondominant deficits. PET showed only subtle decreased metabolism in the left temporal lobe, but SISCOM showed both left and right temporal activity. Amytal testing demonstrated confusion and speech difficulties with both injections, more language difficulties with the right injection. MRI did not show increased signal in either hippocampi. In summary, the patient was suspected to have left temporal lobe epilepsy. However, because there were numerous EEGs that showed right greater than left temporal sharp waves, only subtle PET scan findings, some nonlocalized seizure activity, and no hippocampal sclerosis on MRI, an option for invasive monitoring was offered to the patient and his family. The decision was to proceed with bilateral temporal depths, with a plan to confirm left medial temporal onset prior to resection (Figure 32-4A).
It was unexpected to find the seizure onset at the lateral electrodes, shown on two depth EEG electrode recordings (Figure 32-4B,C). Thus, the invasive data supported a neocortical ictal focus in this patient. However, the depth electrodes evaluated only one point on the brain surface; there was no information about the adjacent cortex. In preparation for a left temporal lobectomy with neocortical resection (and to validate the unusual findings), a craniotomy was performed, and subdural grids were placed (Figure 32-5A,B). The subdural recordings identified the ictal onset at the anteroinferior temporal gyrus, and a standard temporal lobectomy was completed (Figure 32-5C,D). This case does not intend to illustrate an absolute methodology, as some may have chosen to go directly to temporal lobectomy without invasive studies. However, with less than ideal alignment of all data, it illustrates the options available to help decision making.
Figure 32-4.
MRI (axial, proton density-weighted image) showing the location of the left temporal depths. Numbers and arrows illustrate the electrode numbering scheme. Above, next page. EEG from the left depth electrodes in two separately recorded events. In both recordings, the seizure onset occurs in the lateral electrodes with relatively quiet medial electrodes. Red brackets and arrows indicate the earliest onset. Black bracket indicates the second electrode onset.

Figure 32-5.
(A) Lateral and (B) anteroposterior skull radiographs document the placement of two grids and one strip for Case Study 3. The lateral view allows visualization of a large grid over the lateral frontal and temporal lobe surfaces (triangle), a smaller grid under the temporal lobe (open white arrow), and a strip electrode covering the anterior and medial temporal lobe (curved arrow). The anteroposterior view demonstrates excellent coverage of the basal and medial temporal lobe (open black arrow). Skull radiographs are the simplest way to document intracranial electrode placement, although they do not provide detailed information about electrode location relative to the specific brain or lesional anatomy. They can be useful to confirm that no gross movement or shift has occurred if a patient has a violent seizure or accidentally pulls on the electrode leads. EEG recording (C) from the large fronto-temporal grid in case study #3. The focal seizure onset is seen first from electrode pairs 61-62 (black arrow) and 62-63 (red arrow), thus localizing the onset to the region of electrode 62. A basic electrode “map”. (D) serves as a tool to review and analyze electrode pairs with a general representation relative to brain. Again this simple and fast tool does not provide the detailed anatomic correlation available from 2D- or 3D-imaging methods. Electrode 62 is circled in red.
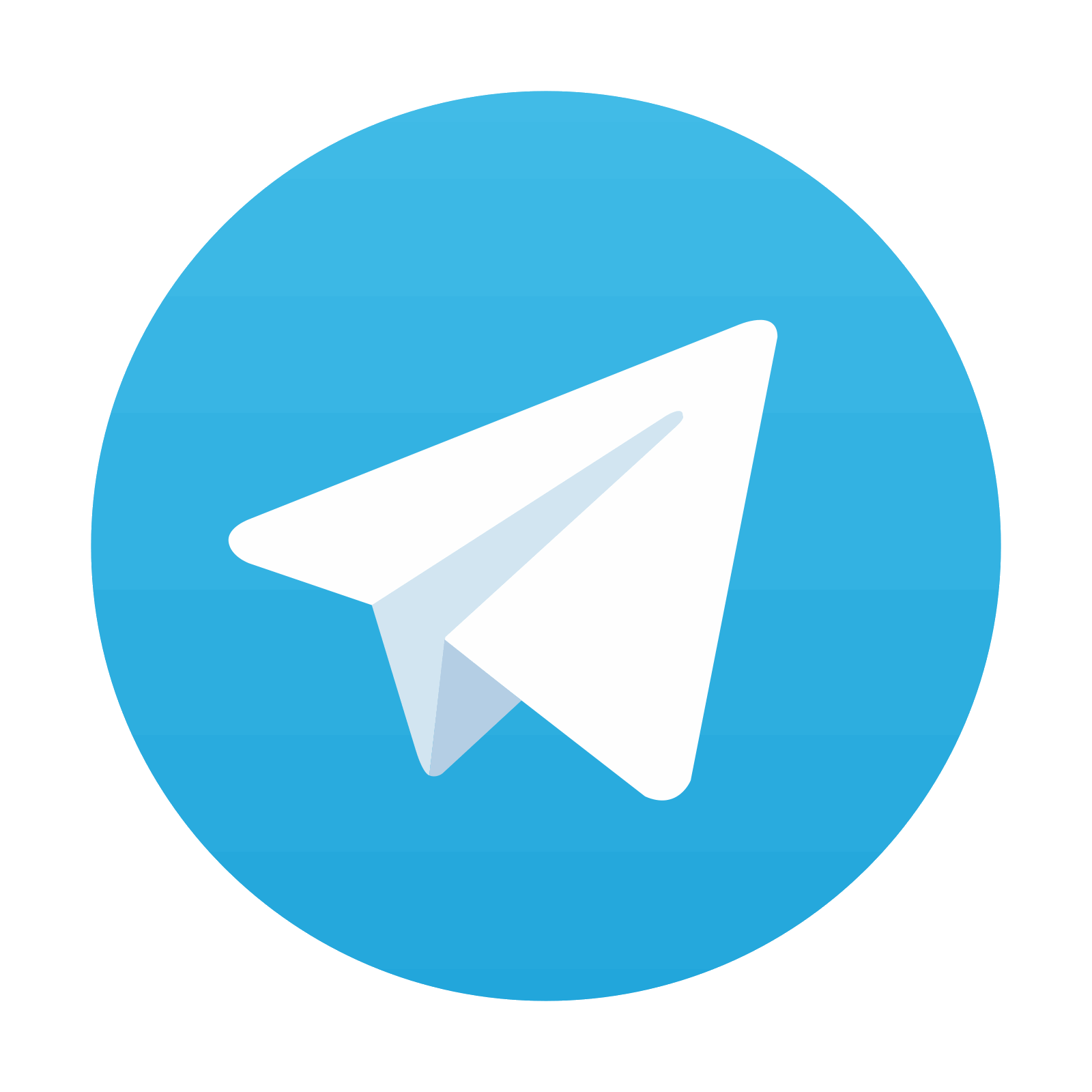
Stay updated, free articles. Join our Telegram channel
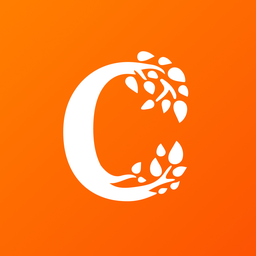
Full access? Get Clinical Tree
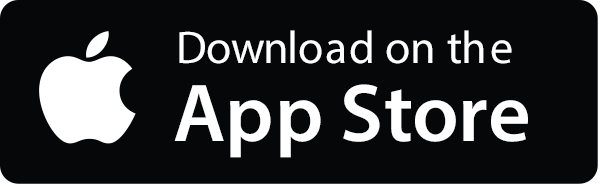
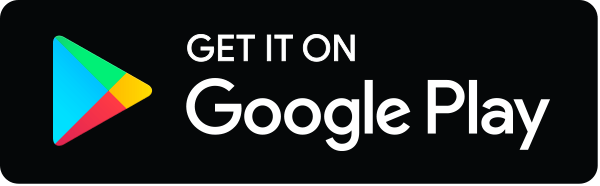
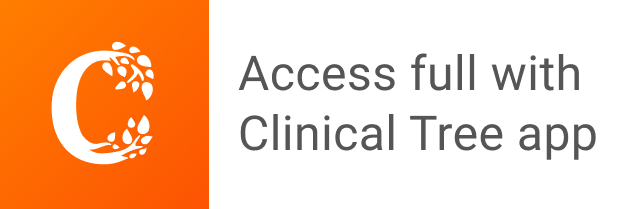