USE OF FUNCTIONAL IMAGING TO STUDY COGNITIVE FUNCTIONS
Madison M Berl and Leigh N Sepeta
Introduction
The use of neuroimaging tools to study the neural correlates of cognitive functioning has become a staple in clinical neuroscience endeavors (Cabeza and Nyberg 2000; Smith et al. 2009). Epilepsy populations are uniquely poised to be a model for comparing how neurological disruption due to a disease disrupts normal development because patients with epilepsy often undergo neuroimaging studies as part of their direct clinical care. Specifically, functional magnetic resonance imaging (fMRI) is a noninvasive method for mapping eloquent cortex and is often an integral step for planning epilepsy surgery (Loring et al. 2014). While the goals of fMRI for presurgical mapping are to map cognitive functions individually in patients with focal epilepsy to identify areas to spare during surgery, this same tool is also valuable in a research context for group comparisons. Moreover, because it is noninvasive, a typically developing control group can be included as a comparison group.
This chapter will address several topics related to functional imaging of cognition in children with epilepsy, including: (1) review of methodological approaches using functional imaging; (2) review by domain of extant literature with summarization of how results have informed us about the functioning of patients with childhood epilepsy; and (3) practical information about strategies for successfully imaging children with epilepsy for clinical studies.
Functional magnetic resonance imaging approaches
Functional neuroimaging has expanded exponentially from the early 1990s, and several design and analysis options exist but, fundamentally, the techniques discussed here rely on the detection of magnetic resonance signal as a result of blood flow over a period of time. The blood-oxygen-level-dependent technique (BOLD) is presumed to be an indirect measure of neural activity.
TASK-BASED ACTIVATION
Seminal fMRI paradigm designs are task based and require active engagement to garner robust activation at the individual level. In epilepsy, language and sensorimotor tasks have been studied the most and are accepted as standard clinical tools for mapping individuals (Woermann et al. 2003; Gaillard 2004; Binder 2011; Bauer et al. 2014). Motor mapping tasks include simple (tongue wiggling, finger tapping, or toe/foot tapping) or more complex tasks (sequence of moves) to activate the motor strip. Simple or complex motor movements can also activate the supplementary motor area. Even imagined movements (e.g. finger tapping) in hemiparetic patients can activate motor cortex (Bartsch et al. 2006). Sensory tasks include sensory stimulation (e.g. brushing of cheek). Also visual tasks, such as a flashing checkerboard, reliably activate primary occipital cortex (Kwong et al. 1992). The range of language paradigms used and the areas they activate have been described extensively elsewhere (Gaillard 2004; Klein et al. 2015). In brief, tasks may be presented in visual or auditory formats and include passive listening to sentences or stories, reading sentences or stories, word generation to semantic or phonemic prompts, sentence judgment, rhyming, and others. Other tasks including object naming, single-word reading, and repetition have not been effective for individual language mapping due to low task burden and/or poor lateralizing effects (Gaillard 2004). Memory tasks have also not been established because of poor activation on an individual basis (Gabrieli et al. 1997; McAndrews 2014). Memory paradigms include word/object recognition, paired associate, scene encoding, and autobiographical (hometown walk) (McAndrews 2014).
Fig. 5.1. Group activation (p < 0.05) from children across four language tasks.
Depending on the area of interest (i.e. temporal/Wernicke’s; frontal/Broca’s; sensorimotor, hippocampus), task selection is based on burdening the area of interest (Fig 5.1). For example, frontal language activation is often an area of interest for presurgical planning; however, choosing a passive listening task may not achieve the goal as a task such as listening to a story does not consistently activate frontal language areas on an individual basis (Berl et al. 2010). As fMRI analysis consists of the comparison of two conditions (e.g. task versus control), another factor in paradigm design is the choice of the control (baseline) condition (Gaillard 2004). For example, language tasks that involve listening to stimuli often have some type of auditory stimuli (e.g. tones, reverse speech) during the baseline condition to remove primary auditory cortex activation, which is bilaterally represented. In doing so, the subtraction yields a lateralized language map, assuming that the patient is lateralized. The drawback is that the subtraction may also remove activation in critical language areas. The clinician/investigator will want to optimize his or her task selection based on the goal for the study.
In addition to paradigm design, data analysis techniques impact findings and conclusions. The issue of which threshold has been discussed in the literature (Seghier 2008) and tools to account for different thresholds are useful (Wilke and Lidzba 2007). Nevertheless, for clinical interpretation, efforts to eliminate spurious activation via rigorous thresholds may then underrepresent neural areas involved in the task. Related to threshold is the decision to conduct whole brain versus region of interest (ROI) analyses. An ROI analysis restricts the number of voxels under consideration, which then lowers the number of comparisons that need to be corrected for to reach significance. While there is strong a priori rationale for particular regions associated with language, motor, and memory, ROI selection is not entirely straightforward. Using a smaller language ROI based on Brodmann’s area 44 or 45 versus a larger ROI based on the entire inferior frontal gyrus is one example. Our group often uses a larger ROI because we want to account for the possibility that a patient has shifted language within the hemisphere and may extend beyond the boundaries of a smaller ROI (Berl et al. 2014). Another aspect of the fMRI data processing pipeline that can impact on activation results is motion correction, which can be particularly challenging in pediatric populations. Postprocessing methods exist to help salvage data (Power et al. 2012; Wilke 2014; NITRC n.d.); however, the best method for ensuring that data do not get lost because of motion is to familiarize the child with the fMRI procedures and practice staying still (Byars et al. 2002; Yerys et al. 2009; Rothman et al. 2016). Activation in terms of extent and magnitude remain core parameters for clinical interpretation of task-based fMRI, but new parameters based on functional connectivity are being explored within active task paradigms (Fig 5.2) (Sepeta et al. 2015). Please see below for more details.
Fig. 5.2. Functional connectivity between specific language regions for children with epilepsy compared to typically developing controls. (*) indicates language connections with a significant difference between groups. IFG, inferior frontal gyrus; MFG, middle frontal gyrus, WA, Wernicke area.
RESTING-STATE (OR TASK-FREE) FUNCTIONAL MAGNETIC RESONANCE IMAGING
Similar to task-based fMRI, resting-state (or task-free) fMRI (rs-fMRI) is based on the BOLD signal, but the participant does not explicitly engage in a task. Functional connectivity is the parameter used to quantify the signal changes across brain regions over time (Friston et al. 1993). Functional connectivity is presumed to represent functional organization of different regions because it consists of simple correlations between regions (Fox and Raichle 2007; Smith et al. 2009). Other methods include effective connectivity, which purports to represent a model of neuronal interaction where one neural system exerts an influence over another (Friston et al. 1997). Different analyses are used with functional and effective connectivity, and include model-independent (e.g. graph analysis, independent components analysis) or model-dependent approaches (e.g. Granger causality, structural equation modeling). Discussion of these methods is not within the scope of this chapter; however, the important point is that different conclusions about how epilepsy affects neuronal networks can be made with different analysis methods.
A potential advantage of rs-fMRI is that several functional networks (e.g. motor, language) may be identified simultaneously (Damoiseaux et al. 2006; Biswal et al. 2010) and the lack of a performance demand might be used in young or low functioning patients who are often precluded from task-based fMRI. In addition, rs-fMRI not only maps functional networks but is promising as a tool to localize seizure focus (Lang et al. 2014). A primary disadvantage of rs-fMRI is that the physiological basis of the resting-state signal is not completely understood and the state is unmonitored; therefore, information about the cognitive processes during the resting state (inner speech, etc.) is not available (for review, see Goodyear et al. 2014). This is important because certain cognitive states may potentially interfere with detecting resting-state networks.
Functional magnetic resonance imaging: Contributions to our understanding of childhood epilepsy
fMRI has enhanced our understanding of how function, particularly language, is represented in epilepsy populations. Language mapping with the same — or developmentally adapted paradigms — yields fundamentally similar activation maps in children as in adults such that typical language is a left-lateralized frontotemporal network (Berl et al. 2014). Nonetheless, fMRI studies also demonstrate how language differs from typically developing controls as well as adults with epilepsy. fMRI studies of language confirm findings from prior studies using invasive mapping techniques that show that atypical language representation occurs in 25–30% of individuals with epilepsy and is associated with clinical factors such as early age at onset (<5 years old), atypical handedness, and developmental pathology (Pujol et al. 1999; Springer et al. 1999; Berl et al. 2005). fMRI studies expanded upon these findings to demonstrate the range of atypical language patterns (Berl et al. 2014). fMRI studies confirmed that atypical activation commonly occurs in homologous regions in the right hemisphere and revealed that cross-dominance is a rare, but existing pattern such that frontal and temporal language dominance are in separate hemispheres (Staudt et al. 2001; Berl et al. 2014b). Moreover, reorganization of only frontal language is associated with specific factors including degree of frontal lobe periventricular white matter injury (Staudt et al. 2001) and atypical handedness (Berl et al. 2014b). fMRI studies with typically developing children inform our understanding of the timing of lateralization such that language becomes more lateralized with age and that temporal regions lateralize earlier than frontal regions (Holland et al. 2007; Berl et al. 2014a), which has implications for the developmental timing of epilepsy surgery and its impact on language functioning. Despite these associations, patients with similar clinical factors do not uniformly shift their language and recent study explores other factors that potentially influence language reorganization such as features of the structures in the contralateral hemisphere (Pahs et al. 2013).
fMRI studies in epilepsy populations have contributed to our ability to predict outcomes. Motor fMRI accurately predicts the location of the motor cortex compared to electrical stimulation (Mehta and Klein 2010) with high sensitivity and specificity and contributes to clinical decision making (De Tiège et al. 2009). Similarly, language fMRI has been found to modify surgical planning and predict postoperative language deficits (Sabsevitz et al. 2003). In adults with temporal lobe epilepsy (TLE), activation in mesial structures predicts memory outcome after temporal lobe resection. Specifically, patients with greater ipsilateral compared to contralateral mesial temporal lobe (MTL) activation on pre-operative fMRI memory testing demonstrate greater memory decline following temporal lobe resection (Jokeit et al. 2001; Rabin 2004; Richardson 2004; Janszky et al. 2005; Richardson et al. 2006; Powell et al. 2007; Bonelli et al. 2010; Negishi et al. 2011). These outcome studies with adults inform models of hippocampal functioning that posit that postoperative memory outcome is predicted by activation in the hippocampus to be resected (hippocampal adequacy) rather than activation in the nonresected hippocampus (hippocampal reserve) (Chelune 1995). Resting-state connectivity is also emerging as a predictor of outcomes in epilepsy populations (Lang et al. 2014). rs-fMRI studies have found that patients who continued to have seizures following surgery had less lateralized functional connectivity than those who were seizure free (Negishi et al. 2011) and the strength of left hippocampus and posterior cingulate functional connectivity was associated with greater decline following left temporal resection (McCormick et al. 2013). Similar to activation studies, these functional connectivity results support the hippocampal adequacy
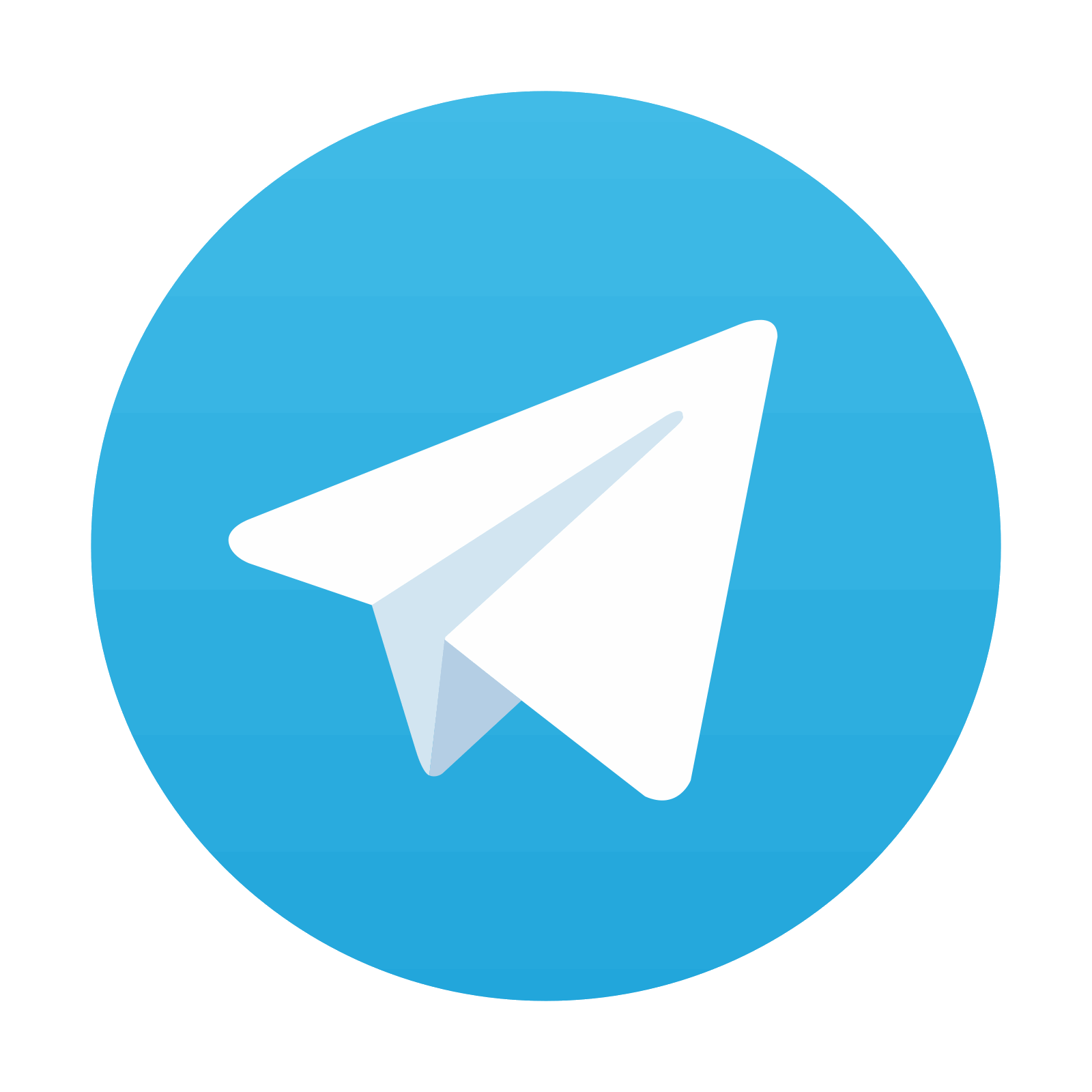
Stay updated, free articles. Join our Telegram channel
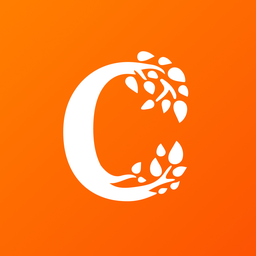
Full access? Get Clinical Tree
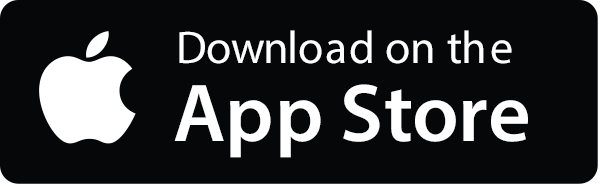
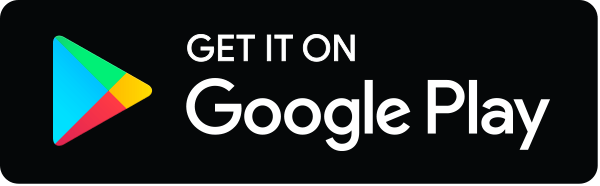