List of abbreviations
SCI
spinal cord injury
CNS
central nervous system
AdV
adenovirus
HSV
herpes simplex virus
LV
lentivirus
AAV
adeno-associated virus
IM
intramuscular
IN
intraneural
IV
intravenous
IT
intrathecal
NGF
nerve growth factor
BDNF
brain-derived neurotrophic factor
NT
neurotrophin
BBB
blood-brain barrier
CSPG
chondroitin sulfate proteoglycan
ChABC
chondroitinase ABC
ADAMTS4
a disintegrin and metalloproteinase with thrombospondin motifs 4
Introduction
Traumatic spinal cord injury (SCI) results from a physical insult to the spinal cord. This leads to disruption of the blood supply and loss of tissue near the site of impact. Following this, there is ongoing detrimental change to the tissue, including inflammation, formation of an inhibitory scar, and inhibition of axonal regeneration and remyelination ( Fig. 1 ). A number of approaches have been tested in pre-clinical models including increasing the intrinsic regenerative ability of neurons or removing the inhibitory molecules present after injury. Approaches include the delivery of neurotrophic factors ( ), strategies to block inhibitory receptor signaling ( ) or using enzymes to degrade inhibitory molecules ( ). However, conventional methods of delivery of such factors have limitations, such as poor stability in vivo, poor tissue penetration, and the potential for off-target effects ( ). Viral vector gene therapy can be applied to a number of these approaches to help address these issues. This chapter describes different viral vectors that have been used to target the spinal cord, different delivery methods, and examples of how viral gene therapy has been used to effectively target therapeutic agents to the spinal cord.

Viral vector approaches
Viral vectors allow for long-term, stable, and targeted gene expression. A number of viral vectors have been trialed as a gene therapy approach to SCI repair. Each of these approaches has advantages and shortcomings ( Fig. 2 ).

Adenovirus
Adenoviruses (AdVs) are encapsulated double-stranded DNA viruses with a 36 kb genome and therefore have the capability of holding large transgenes. They can transduce both dividing and non-dividing cells and exhibit high transduction efficiency in a wide variety of cell types. The genome remains extra chromosomal in the nucleus, thereby the transgene is not integrated into the host cell’s genome, avoiding risk of tumorigenesis. However, transgene expression is only transient and expression is lost during division as the non-integrated genome is received by only one daughter cell. Also, an immune response is elicited against AdV viral antigens leading to inflammation and limiting the period of transgene expression ( ).
Poliovirus
Polioviruses are small, non-enveloped RNA viruses and recombinant poliovirus replicons can be used for gene therapy ( ). As infection is restricted to motor neurons in the hind brain and spinal cord these have been suggested as a potential vector for use in the spinal cord ( ). Advantages of poliovirus include that its RNA genome is replicated in the cytoplasm, leading to very high levels of gene expression ( ). Multiple inoculations of poliovirus replicons in the spinal cord has been shown to be safe, give good levels of gene expression with no functional deficits or inflammatory response. However, studies show that gene expression is not sustained ( ), although this could be of benefit if transient gene expression is required.
Herpes simplex virus
Herpes simplex virus (HSV) is an enveloped virus that contains a large (150 kb) double-stranded genome, allowing for an almost unlimited packaging capacity for transgenes. They demonstrate an exceptional capability to provide long-lived infections, with the genome being maintained extra-chromosomally in the nucleus of host cells ( ). Advantages of HSV are it is neurotrophic and has a tendency to remain latent while permanently transducing target cells. The large size of the vector allows for expression of multiple genes which has been suggested as advantageous in development of effective treatments ( ). However, following transduction a number of viral proteins are expressed, leading to cytotoxicity and immune responses against targeted cells ( ).
Lentivirus
Lentiviral vectors (LV) are a sub-class of retroviruses derived from human immunodeficiency viruses. Due to their ability to transduce dividing and non-dividing cells, LV vectors can transduce post-mitotic neurons, making them a powerful tool for targeting the CNS ( ) and as such have been used extensively for gene therapy targeting of the spinal cord ( ). Because LV genomes are integrated into the host genome they can provide long-term stable gene expression. While integration poses a risk of oncogenic transformation it has been suggested that this is reduced by the virus integrating into gene ends rather than promoter regions ( ).
Adeno-associated virus
One of the most well-characterized vectors for gene therapy is derived from adeno-associated virus (AAV). Wild type AAVs are small, 4.7 kb, linear, single-stranded DNA (ssDNA) viruses in the Parvovirus family. For therapeutic use, the rep and cap genes, involved in viral replication and capsid production, respectively, are removed and replaced by an expression cassette containing therapeutic transgenes under the control of a promoter and flanked by the AAV ITRs, required for directing genome replication and packaging, forming what is termed a recombinant AAV (rAAV) ( ). AAVs are considered ideal for gene therapy approaches as they are small and non-replicative, can transduce dividing and non-dividing cells, are non-pathogenic to humans and can provide long-lasting changes in gene expression. A disadvantage of AAV vectors is their small genome, impacting on the size of transgenes that can be used. A large number of clinical trials using AAV have demonstrated the relative safety of AAV gene therapy ( ).
Routes of administration
A number of different routes of administration are available for gene therapy delivery to the spinal cord ( Fig. 3 ) and are classified as either remote or direct delivery ( ). Remote delivery involves non-invasive or minimally invasive routes of administration such as intramuscular (IM), intraneural (IN), intravenous (IV), and intrathecal (IT). These are dependent on the capability of specific gene therapy serotypes to travel to the spinal cord following peripheral delivery. Direct delivery involves an invasive surgical approach that directly targets a specific area of the spinal cord tissue via injection of the gene therapy and is referred to as intraparenchymal delivery.

Intramuscular and intraneural delivery
Retrograde transport is the transport of viral vector particles to neuronal cell bodies via axonal transport following IM or IN (e.g., sciatic nerve) injection. A number of viral gene therapies can be transported to the spinal cord from peripheral injection sites such as skin, muscle, or peripheral nerves ( ). Following injection of an HSV gene therapy into the gastrocnemius muscle of the rat, gene expression in neurons of the anterior horn spreads widely through the spinal cord and has been shown to be maintained for up to 182 days post-injection ( ; ). Adenovirus has been used successfully in a number of studies to target the spinal cord via IM injection and delivery of neuroprotective compounds has been achieved ( ; ). A number of AAV vector serotypes have been demonstrated to transduce neurons of the spinal cord following both IM and IN injection [reviewed in ( ) ( ; )]. However, retrograde transport of AAV vectors can have low efficiency. To overcome this, targeted evolution can be used to improve transport ( ; ). When Tet1, a peptide with high affinity for the tetanus toxin GT1b receptor that undergoes retrograde transport in the spinal cord, was grafted onto AAV1, enhanced axonal terminal binding and uptake was seen, with this vector showing a four-fold enhancement in retrograde transport in DRG explants ( ). While IM injection is relatively easy and non-invasive, this largely restricts transduction to motor neurons or other populations in the anterior horn and so is not able to provide a more diffuse treatment. Also, the large amount of vector that would be required to transduce larger human muscles and the relatively long axons compared to rodents has been raised as challenges to this approach for vector delivery.
Intravenous delivery
A number of AAV vectors, especially AAV9 and similar serotypes have been found capable of crossing the blood-brain barrier and shown promise for delivering therapeutic agents to the whole spinal cord ( ). However, systemic delivery using AAV leads to transduction of peripheral organs and there can be reduced levels of transduction due to the presence of antibodies against the virus. Questions also remain as to whether results seen in animal models will translate to humans. A vector, AAV-PHP, was found to have better transduction of the CNS after IV injection than its parent AAV9 but its ability to cross the BBB has been found to be species and even mouse strain dependent due to lack of its receptor (Ly6A) ( ). No known analogue for this receptor is found in humans and such species differences may impact on the usefulness of such novel BBB-crossing AAV capsids. Also, while whole spinal cord transduction may be useful for developmental disorders, targeted transduction is likely to be of more use to treat SCI.
Intrathecal delivery
This approach allows for widespread transduction, while avoiding off-target effects ( ). The vector is delivered into CSF, via the sub-arachnoid space, allowing perfusion into the spinal cord tissue. Gene therapy vectors including AAV ( ), adenovirus ( ), lentivirus ( ), herpesvirus ( ) and poliovirus ( ) have all been used successfully to target the injured spinal cord and delivery therapeutic agents. While intrathecal injections result in greater spatial distribution, the trade-off is a comparatively lower level of expression compared to delivery into the tissue ( ).
Intraparenchymal delivery
Intraparenchymal injection of viral vectors into the spinal cord of animal models has been widely explored and used successfully by a number of groups ( ). This allows for focal targeting of a vector but bio-distribution can be increased by multi-level injections to the cord. Intraparenchymal injection allows for much lower volumes of virus to be used compared to remote delivery techniques. However, a major barrier toward clinical translation is the validation of a safe surgical approach for delivery to the spinal cord in large animal models and humans. Pre-clinical studies have shown the safety of delivering AAV to the cervical spinal cord of pigs ( ), so this does appear to be a feasible approach.
Viral vector approaches for therapeutic delivery
Neurotrophins
Following SCI, axons fail to regenerate, at least in part due to low intrinsic regenerative capacity. This has led to a large field of study testing the use of neurotrophic factors, including nerve growth factor (NGF), brain-derived neurotrophic factor (BDNF), and the neurotrophin family (NT-3, NT-4/5), to create a growth permissive environment after an injury ( ). However, delivery of neurotrophic factors to the spinal cord has major limitations. Their short half-life and issues with crossing the BBB makes it difficult to deliver biologically relevant amounts to the spinal cord for prolonged periods of time. Also, widespread delivery of neurotrophins can lead to adverse effects ( ). Viral vector delivery has been used to target neurotrophins to specific neuronal and axonal populations, either directly to the cord for expression from endogenous cells or via viral transduction of cells that are then transplanted into the cord. This has been used successfully to promote axonal regeneration and is extensively reviewed elsewhere ( ; ; ; ; ).
While axonal regrowth can be promoted by the presence of neurotrophic factors, growth beyond the spinal cord lesion site is often limited due to continual presence of growth factors and the absence of a gradient of these factors across the injury site ( ). The use of gene therapy approaches has helped overcome some of these issues through allowing for spatial and temporal regulation of neurotrophin delivery. Viral gene transfer has been used successfully to establish neurotrophin gradients. Viral delivery of NT3 leads to growth of axons through a spinal cord lesion ( ; ) and a gradient established by lentiviral BDNF delivery promoted axonal sprouting toward the highest concentration of BDNF ( ). A study by ( ) using a tetracycline-regulatable retroviral system to control BDNF expression in transplanted fibroblasts, showed that only transient expression was required to induce axonal growth into the injury site. Viral vectors allow for the targeting of specific cell populations. Targeted evolution of AAV vectors has been successful in promoting specific cell tropism ( ). As different neurotrophins stimulate different neuronal and axonal populations, the use of engineered AAV viral vectors in conjunction with cell specific promoters would allow for specific targeting of the correct cell type and would help prevent adverse effects caused by widespread neurotrophin delivery.
CSPG
Another major hurdle preventing axon regeneration and repair after SCI is the release of growth inhibitory molecules; myelin-associated growth inhibitors, chemo-repulsive guidance molecules, and highly sulfated proteoglycans, especially chondroitin sulfate proteoglycans (CSPGs) ( ; ; ). Following CNS injury, CSPGs are rapidly upregulated at the lesion site by reactive astrocytes within the glial scar ( ) and form a chemical barrier for axon regeneration via interaction of the sugar GAG side chains with a number of receptor proteins (reviewed in ( ).
Degradation of CSPGs to overcome this inhibition is being trialed as a therapeutic intervention for SCI. Chondroitinase ABC (ChABC) is a bacterial enzyme that degrades the GAG side-chains of CSPGs. In vivo delivery of this enzyme leads to beneficial effects in a number of experimental SCI models ( ) although results have been mixed, depending on the type of injury ( ). This is thought to be at least in part due to issues with enzyme stability ( ). Therefore, gene therapy approaches where cells are transduced with a ChABC viral vector have been tested as a promising tool for achieving stable delivery of the enzyme. Early attempts using gene therapy had limited success in digesting CSPGs ( ; ; ). However, modification of ChABC through mutagenesis of key N -glycosylation sites allowed the expression and efficient secretion of active ChABC enzyme by mammalian cells ( ) and a number of studies have successfully shown that lentiviral delivery of mammalianized ChABC leads to improvement in both cervical and thoracic models of SCI, reduced lesion size, improved sensorimotor function (sensory axon conduction, hindlimb function, forelimb reaching, and grasping), increased axonal conduction, and increased serotonergic and vGlut1innervation ( ; ; ; ; ). While to date gene therapy approaches to deliver ChABC have not been trialed in non-rodent species, promising results in large animal models following delivery of ChABC enzyme to the injured cord have been seen. Injection of ChABC into the primate spinal cord following a C7 transection led to improved hand function ( ) and a recent clinical trial using ChABC injection in canines with chronic, naturally occurring spinal cord injuries showed improved forelimb-hindlimb coordination ( ). This suggests that the use of gene delivery of ChABC has potential as a clinical therapy.
It is now well-understood that ECM components, including CSPGs, impact on the inflammatory response following SCI ( ). A number of studies have shown that a lentiviral approach to delivering ChABC, as well as causing extensive remodeling of the extracellular matrix and axonal regeneration, is able to modulate the post-injury immune response ( Fig. 4 ). A study by ( ) using lentiviral delivery of ChABC in a rat thoracic contusion model examined the expression profile of M1/M2 macrophage polarization markers. This study found that ChABC treatment had a distinct effect on the immune signature in the injured tissue, increasing expression of the pro-regenerative cytokine IL-10, with a corresponding reduction in the pro-inflammatory cytokine IL-12B. This demonstrates a distinct, ChABC-mediated anti-inflammatory response following SCI associated with large-scale removal of CSPGs. Lentiviral ChABC delivery leads to an increase in the levels of CD68 and CD206 ( ). These are markers of the M2 macrophage phenotype, which promote resolution of inflammation, enhance phagocytosis, angiogenesis, and tissue remodeling following injury ( ).
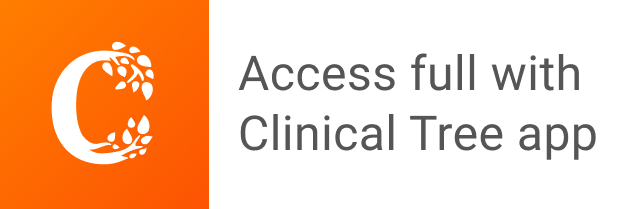