Chapter 2
What Happens to the Upper Limb After Brain Injury?
Chapter objectives
- Provide an overview of the neural and non-neural components of the motor system that contribute to upper limb movement.
- Describe the underlying causes of the upper motor neuron syndrome.
- Discuss the changes that occur in the upper limb due to the upper motor neuron syndrome.
- Consolidate learning about the influences of the upper motor neuron syndrome on the upper limb through clinical examples.
Abbreviations
ASP | Arm Spasticity Patterns |
BoNT-A | Botulinum neurotoxin-A |
CMC | Carpometacarpal (joint) |
CNS | Central Nervous System |
DIP | Distal interphalangeal (joint) |
EMG | Electromyography, electromyographic |
FCU | Flexor Carpi Ulnaris |
GMFCS | Gross Motor Functional Classification System |
GT | Gschwind and Tonkin forearm classification |
HGF | House, Gwathmey and Fidler thumb classification |
HIPM | Hypertonicity Intervention Planning Model |
IP | Interphalangeal (joint) |
MC | Metacarpal (bone) |
MCP | Metacarpophalangeal (joint) |
PIP | Proximal interphalangeal (joint) |
UMN(s) | Upper motor neuron(s) |
UMNS | Upper motor neuron syndrome |
Z&Z | Zancolli and Zancolli hand classification |
2.1 Motor control and normal movement
Motor control involves the process of planning, initiating, organising and completing movements that are appropriate for each activity and task environment. The capacity to adapt movement to suit a variety of situations requires cooperation between different systems within the person, such as the sensory/perceptual, cognitive and neuromuscular systems. It also requires information processing across different levels of the central nervous system (CNS). For example, sometimes movement is fast, automatic and involuntary, involving only neuromuscular systems, such as when one withdraws the hand quickly after touching a hot surface. This automatic reflex movement involves neural connections between sensory input and motor output at a spinal cord level only (although the connections may involve interneurons and one or more spinal circuit segments). At other times, such as when learning a new skill, movement will be deliberate and more consciously controlled as cognitive/memory systems interact with sensory/perceptual and neuromuscular systems to make fine adjustments to motor output. This type of movement involves information processing across different systems, and between the cortical levels of the CNS involved in planning and programming movement, and the spinal levels involved in executing the movement [1–3].
For normal upper limb movement to occur, all components of the motor system (neural, muscular and skeletal) need to be intact and able to respond effectively to motor commands and activity requirements. The focus of this text is the upper limb and, in particular, promoting arm and hand function after brain injury. Therefore, this chapter provides a relatively brief overview of only those components of the motor system most involved in effecting the ‘mechanics’ of upper limb movement (such as the descending tracts, the spinal cord and its circuits, muscles and connective tissues). The ‘cognitive’ aspects of motor control (such as motor learning or movement initiation) are not detailed, nor are the many linkages between sensory/perceptual and motor systems, which are covered in other texts [4–6].
2.1.1 Neural components of the motor system
The neural components of the motor system include the spinal cord, brainstem, descending pathways, motor cortex (primary motor, premotor and supplementary) and the association cortex (prefrontal and posterior parietal). These components can be described as being organised into ‘lower’ and ‘higher’ levels in terms of their responsibilities for the different aspects of movement control (see Box 2.1). While ‘lower’ level components have more direct control over muscles, the ‘higher’ level components have responsibility for more abstract and complex aspects of movement control. However, despite each component having different responsibilities within the motor system, they interact and cooperate with one another to produce coordinated movement.
The spinal cord and brainstem are involved in controlling movements through automatic, reflex activity. For example, the spinal cord controls the speed and force of muscle contraction through reflex action and, similarly, the brainstem is concerned with maintaining balance and posture. Local brain stem and spinal cord circuits contain the cell bodies of the lower motor neurons which send out their axons to control skeletal muscles in the head and body, respectively. Descending pathways form the third component of the motor system and are comprised of the axons of upper motor neurons (UMNs), that is, neurons whose cell bodies are located either in the cortex or the brainstem. The role of the UMNs is to regulate the excitability of the lower motor neurons, either directly, or indirectly via interneurons. In contrast to the reflex activity of the ‘lower’ components of the motor system, the motor cortex and association cortex areas are involved in planning, initiating and coordinating skilled, voluntary movements.
While not structurally a part of the motor or association cortices, the basal ganglia and cerebellum are functionally connected with the motor system through their influence on the descending pathways. The basal ganglia are three interconnected groups of neurons (the caudate nucleus, putamen and globus pallidus) concerned with selecting and initiating voluntary movement, and with suppressing unwanted movement. The cerebellum is involved in detecting errors between planned and actual movements [9, 10]. Table 2.1 lists the neural components of the motor system together with the motor disorders that are commonly attributed to each following brain injury.
Table 2.1 Neural components of the motor system: responsibilities and disorders [9–11].
Component | Motor responsibilities | Motor disorders due to damage at component level |
Spinal cord |
|
|
Brainstem |
|
|
Cerebellum |
|
|
Basal ganglia |
|
|
Thalamus |
|
|
Primary motor cortex |
|
|
Premotor cortex |
|
|
Supplementary cortex |
|
|
Association cortex (prefrontal, posterior parietal) |
|
|
Primary somatosensory cortex |
|
|
Descending motor pathways |
|
|
2.1.2 Non-neural components of the motor system
The musculoskeletal system forms the non-neural component of the motor system and includes muscles, connective tissues, bones and joints. The functions and properties of muscle (including muscle tone) and connective tissues are described in this section.
2.1.2.1 Skeletal muscle: excitability and contraction
The main function of skeletal muscle is to provide movement, which is facilitated through its properties of excitability, contractility, elasticity and extensibility (see Box 2.2). Excitability and contractility (capacity for contraction) are described in this section. Skeletal muscles and connective tissues share the properties of elasticity and extensibility which are addressed in Section 2.1.2.3.
Skeletal muscles facilitate limb movement by adjusting their length and tension through the contraction of muscle fibres which are organised into motor units. Each motor unit consists of a single alpha motor neuron (with its cell body located in either the brainstem or ventral horn of the spinal cord), its axon (the long fibre of the neuron that conducts nerve impulses and synapses with the muscle fibre at the neuromuscular junction), and the muscle fibres that it innervates. The muscle fibres within each motor unit are of the same metabolic type, and the number of fibres associated with each motor unit depends on where the muscle is located and the nature of its action. For example, in the hand where finer movements are required, a motor unit will be associated with fewer fibres (it is a small motor unit) and will generate less force, while in a large, more powerful muscle it may be associated with thousands of fibres (a large motor unit) and will generate greater force on contraction [8].
There are different ways of classifying motor units. A common approach is to classify them according to the speed of contraction (or twitch) and the fatigability (or endurance) of their muscle fibres (Table 2.2). Classified in this way, there are three motor unit types: type 1 or slow-contracting, slow-fatigable (S), type 2A or fast-contracting, fatigue-resistant (FR), and type 2B/2X or fast-contracting, fast-fatigable (FF) [14–17].
Table 2.2 Characteristics of motor units and muscle fibres [9, 14–16].
Characteristic | Motor unit | ||
Type 1 Slow-contracting, slow-fatigable (S) | Type 2A Fast-contracting, fatigue-resistant (FR) | Type 2B/2X Fast-contracting, fast-fatigable (FF) | |
Muscle fibre, axon, motor neuron | Small muscle fibre, slow conducting axon, small motor neuron | Large muscle fibres, fast conducting axons, large motor neurons | |
Contraction speed | Slow | Moderately fast | Moderate |
Fatigue resistance | High | Moderate | Low |
Power or force | Low | Moderate-high | High |
Recruitment | First | Second | Last |
Activity type | Sustained effort (standing, long-distance running) | Sustained effort, high power output (middle-distance running, swimming) | Brief, intense effort (weightlifting, sprinting) |
The production of muscle force depends on a variety of neuromuscular factors (see Table 2.3), including the number and type of motor units recruited in a contraction and their firing rates. Muscle strength or force increases when the number and/or the firing rates of already-activated motor units increases. Changes in firing rates allow adjustment of force production, with different motor units having an optimal range of firing over which tension increases. An orderly recruitment pattern also occurs according to the amount of force required for task completion. Low force-producing motor units are recruited first (type 1), with higher force-producing motor units recruited as necessary (type 2A then type 2B/2X). Thus, the succession of recruitment of motor units provides a smooth increase in tension development [18, 19]. In general, the muscles of the upper limbs contain more type 2B than other types of fibres, at least in their superficial areas, with an increase in type 1 fibres in their deeper areas. This means that the muscles are able to respond with fast contraction, which is then sustained by recruitment of less fatigable fibres [20].
Table 2.3 Neuromuscular factors influencing muscle force production [25–28].
Factor | Influences on force production |
Motor unit |
|
Sarcomere (contractile factor) |
|
Fascicleb length |
|
Muscle bellyc length |
|
Anatomical cross-sectional area (ACSA) |
|
Physiological cross-sectional area (PCSA) |
|
Muscle thickness |
|
Fascicle angle |
|
Pennate angle |
|
Tendon length and compliance |
|
a See Figure 2.1.
b Fascicles are groups of muscle fibres.
c Muscle belly is the length of the whole muscle (i.e. many fascicles).
Sarcomeres are the contractile part of muscle, found within the muscle fibres and grouped together into fascicles. Each sarcomere is made up of two types of overlapping muscle proteins or filaments: myosin (thick filaments) and actin (thin filaments). During contraction, the actin filaments slide toward one another past the myosin filaments, breaking and reforming cross-bridges in a process called cross-bridge attachment (see Figure 2.1). The amount of tension that can be generated by a muscle during contraction depends on the number of cross-bridges that form, or alternately, the amount of overlap between the actin and myosin filaments. The best position for the muscle to develop maximal contraction and force generation (that is, the optimal range of overlap for the filaments), is when the muscle is at its resting length. If the muscle is held in a shortened position, then the thin actin filaments are already close together, and are unable to overlap the thick myosin filaments any further because the overlapping zone is already as large as possible. If the muscle is held in a lengthened position the thick and thin filaments may be too far apart to and the zone of overlap will be small. In both these situations the potential of the muscle to contract and generate force is reduced. Skeletal muscle cells can contract until they shorten by about 30% [21].
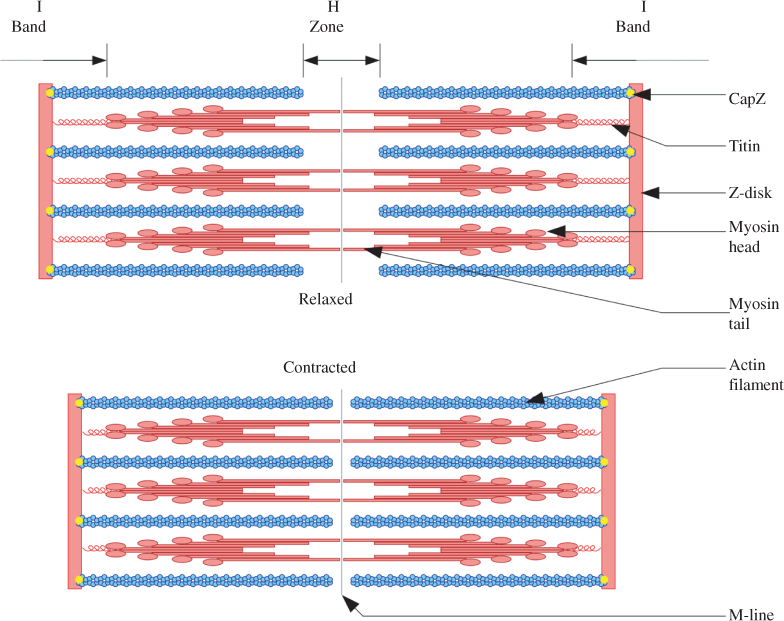
Figure 2.1 Sliding filament model of muscle contraction. Actin filaments: thin strands of protein in a sarcomere; CapZ: protein that caps the end of the actin filament, located in the Z-disk; H-zone: region in which only the thick mysosin filaments are present; I-band: lighter region in the sarcomere that contains only the thin actin filaments; M-line: supporting proteins located in the middle of the sarcomere’s H-zone, and which hold the myosin filaments together; Myosin; thick bipolar strand of protein; Titin: elasticated protein; Z-disk, Z-line: delineate each end of a sarcomere.
Titin (or connectin) is another protein molecule found in the sarcomere. It is a long, coiled molecule that is wrapped around the myosin filaments and attached to the Z-line that forms the borders of each sarcomere. Titin behaves like a spring, returning the sarcomere to its original length following stretch [22, 23]. It has been suggested that different muscle groups may contain different forms of titin which influence the tension and elastic limits within sarcomeres, meaning that some muscles may have more capacity for stretch or extensibility than others [24].
2.1.2.2 Connective tissue: strength and flexibility
Ligaments, tendons, joint capsules, tendon sheaths, cartilage and other related structures are collectively described as connective or fascial tissues. The structural fibres of connective tissues (collagen, elastin and fibrin) are held together by chemical bonds and are surrounded by a filler gel that lubricates the fibres. Collagen is a fibrous protein that gives connective tissues their strength and flexibility. Connective tissues differ in terms of the type (more than 20 types of collagen have been identified), amount, density and alignment of their collagen fibres [29]. For example, the collagen fibres of dense connective tissues (bone, tendons and ligaments) are tightly packed and mostly aligned in one direction. In contrast, loose connective tissues (in muscle, joint capsules and fascia) have irregular or crisscrossed collagen fibre alignment and are more flexible [30, 31].
Tendons attach muscle to bone and transmit mechanical forces through the muscle–tendon unit, allowing for joint movement [32]. They are integrated into the neuromuscular system through sensory receptors (Golgi tendon organs) that are embedded in the muscle–tendon junction, sending information about changes in muscle tension to the CNS [8, 33]. Ligaments connect bones or cartilage to one another and, together with joint capsules, provide stability to joints [30, 34].
Articular or hyaline cartilage is another form of connective tissue. It is flexible and functions to distribute loads within the joint and to minimise friction between articulating joint surfaces. Cartilage differs from other connective tissues in that it does not contain blood vessels. This means that it grows more slowly and is also slower to repair itself if damaged [35].
Bone is a specialised connective tissue that contains tightly packed, parallel collagen fibres and inorganic minerals (mainly calcium phosphate) which provide rigidity. Bone has many blood vessels and is therefore able to repair itself relatively quickly. It is also highly adaptable to the mechanical demands that are placed on it. For example, changes in density are common in response to either disuse or increased use [34, 36].
2.1.2.3 Muscle and connective tissue: elastic, plastic, viscous and viscoelastic properties
Soft tissues show different responses to various deforming forces, whether compressive, tensile (stretch) or shear (sliding). The different responses to such forces include elasticity, plasticity, viscosity and viscoelasticity (see Box 2.3) [12, 30].
Muscles and tendons are both characterised by elasticity, although this is due to different mechanisms. The elastic property of tendons is mainly due to the ‘crimped’ (zigzag, or wavy) structure of their collagen fibrils, which straighten out as tension is applied [22, 32]. The elasticity of muscles is thought to be due to several possible mechanisms. These include (i) the small amount of the protein elastin in the intramuscular connective tissue that surrounds muscle fibres, (ii) weakly formed actin and myosin cross-bridges in the contractile sarcomere and (iii) the coiled protein, titin, also found in the sarcomere (see Section 2.1.2.1). Titin is described as the structure most likely to be responsible for muscle’s elastic response [22, 23].
In addition to their elastic properties, connective tissues display viscoelasticity when their load (or stretch) is maintained. This is related to the combination of their elastic properties and their fluid or gel components (these components are viscous, having a glutinous consistency). When connective tissues are subjected to a slow stretch and are then held at their new length with the joint at a stable angle, resistance in the tissues gradually reduces to a steady level (this reduction is called stress relaxation), and less force is needed to hold the joint angle stable. If the tissues are subjected to a slow but constant force (torque) during stretch, the tissues will continue to elongate (this elongation is called creep) and the joint angle increases [12, 22]. When resting muscle is subjected to small, low-load passive stretch it shows a very high resistance to that stretch, called short range stiffness. The bonds between myosin and actin filaments are proposed to be responsible for this stiffness [37]. However, if the muscle is held in a constant, stable position, the resistance reduces and stress relaxation will occur as described above for connective tissues. If, after stretch and viscous deformation (creep), the muscle is shortened and returned to its original position at which the stretch was begun, resistance will be found to have returned as well. This return of resistance is called stress recovery [12, 22].
2.1.2.4 Muscle tone
Muscle tone is described as the constant state of mild tension observed in muscles at rest. Clinically, normal muscle tone is identified as the slight resistance or stiffness that is felt in the muscles when the limb is moved passively at a slow, consistent rate of movement (between 2° and 12° per second) [40]. Normal muscle tone enables effective stabilisation of joints by balancing the tension in opposing muscle groups around a joint. Normal postural muscle tone assists in maintaining body posture with minimal energy costs [8, 38].
Historically, normal resting muscle tone has been described as the result of a constant, small amount of muscle contraction (due to the stretch reflex). However, no electromyographic (EMG) activity is typically found in normal muscles at rest or during slow passive stretch (the muscles are EMG-silent). Normal muscle tone, therefore, appears to be due to the intrinsic, passive rheologic (resistive) properties of the soft tissues. as described in Section 2.1.2.3 and Box 2.3. That is, the resistance or stiffness felt when ranging a relaxed limb that is not affected by brain injury is due to characteristics such as the elasticity and viscoelasticity of the surrounding connective tissues and muscle fibres, and the actin and myosin cross-bridges formed in the resting muscle [40]. Simons and Mense [41] have therefore defined normal resting muscle tone as “elastic and/or viscoelastic stiffness in the absence of contractile activity (motor unit activity)” (p. 3).
2.2 Upper limb movement
The upper limb is involved in a variety of functions during daily activities. Depending on the context and the task, the arm and hand are involved in reach, grasp and manipulation, transporting, lifting, assisting in balance and, on occasion, providing support for the body [42]. Vision, postural stability (at the trunk and pelvis) and shoulder stability and movement (at the scapula and clavicle), are all important components that support placement of the arm and hand for reach and grasp [43]. The trajectory, speed and orientation of the arm and hand during movement are all influenced by the nature of the reaching task, the type, size and orientation of the object being grasped, the position of the person (for example, sitting or standing), and the context in which the task is occurring. Although effective upper limb movement requires many joints and muscles to work together, the CNS ‘simplifies’ control of such movement through the use of ‘synergies’, that is, by coupling or linking movement segments together so that less effort is expended on controlling each aspect of commonly used movements [44, 45].
2.2.1 Reaching to grasp
Reaching to grasp is divided into three phases, namely, transport, grasp and manipulation [46]. The transportation phase involves positioning and moving the limb towards an object and is quicker than the grasping phase, when the final finger and thumb adjustments are made for picking up the object. Nevertheless, preparing for grasp begins at the start of the transport phase, with hand opening (or aperture) at its widest as the transport phase slows at the end of the reaching movement. As the hand nears the object to be grasped, stability is provided by wrist extension and the index finger initiates hand closing. During reaching, the thumb is positioned in a stable abducted and extended position, possibly allowing visual monitoring of the size of the grasp aperture. If sensation is impaired, the hand aperture is wider than usual and a more conservative grasp (for example, a whole-hand grasp rather than a finger-tip grasp) seems to be chosen [46, 47].
Reach and grasp may be achieved using either one limb or both together. When undertaking a bimanual (two-handed) task, both limbs may be doing the same action (for example, holding the steering wheel when driving) or different actions (such as, when driving, using one hand to hold the steering wheel while the other manipulates the gear stick). Two-handed tasks are therefore associated with complex spatiotemporal coordination and organisation [42].
2.2.2 Grasp and manipulation
The next phase of reaching to grasp involves handling and controlling the grasped object. Coordinated grasp and manipulation (or prehension) require the shoulder, elbow, forearm, wrist, fingers and thumb to move into, and maintain, a variety of positions, depending on the task demands [46]. Thus, grasping requires a blend of mobility and stability across the forearm, wrist, and in the three structural arches of the hand [46] (Figure 2.2). The distal transverse arch is oblique, rigid at the index and middle finger metacarpophalangeal (MCP) joints, and mobile at the thumb, ring and little finger MCP joints. The third metacarpal head (middle finger) is slightly higher on the dorsal surface of the hand than those of the second (index) and fourth (ring) fingers; the metacarpal head of the little finger drops away from that of the ring finger. In addition, the metacarpals become slightly shorter from the index finger towards the little finger, creating an oblique angle from the radial to the ulnar border of the hand. Positioning that does not maintain the distal transverse arch impairs cupping of the palm for grasp and also the ability of the thumb to oppose the fingers (see Figures 7.2, 7.9 and 7.14).
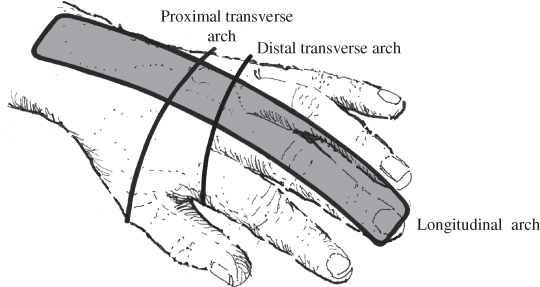
Figure 2.2 Arches of the hand at rest [48, 49].
The longitudinal arch of the hand is mobile and follows the long lines of the metacarpal and phalangeal bones. It allows flexion of the MCP, proximal interphalangeal (PIP) and distal interphalangeal (DIP) joints. The thumb, ring and little finger metacarpals move to accommodate different sized objects in the palm. They are able to stabilise objects against the rigid portion of the arch provided by the index and middle finger metacarpals. The proximal transverse arch is located at the level of the distal carpal bones. It is rigid and provides mechanical advantage to the tendons of the finger flexor muscles by acting as a fulcrum for their movement [48].
The hand is able to achieve a number of different types of grasps, or grips, including power and precision grips (Table 2.4). A power grip involves the whole hand, while a precision grip involves different positions and combinations of the thumb and fingers, and possibly other parts of the hand such as the thumb web-space or the ulnar side of the hand for stabilising objects [50]. The typical hand position for a power grip is between 10° and 30° of wrist extension, finger MCP flexion to approximately 90°, and finger PIP joint flexion between 45° and 60°. The thumb is held abducted and rotated at the carpometacarpal (CMC) joint so that the thumb tip is opposed to the fingers, with its pad in line with those of the fingers (Figure 2.3) [49]. During precision grips, the thumb is often adducted and rotated at the CMC joint to bring the pad across the palm to oppose either the index finger pad by itself (for example, to pick up a pin), or all the finger pads together (such as when picking up a small ball). The fingers may also curl around an object in different configurations (for example, when holding a carving knife or a teacup handle), with the palm and the thumb providing stability [50]. Grasp stability depends on the weight and surface characteristics of the object, and whether the object is solid (e.g. a ball) or moves and changes shape (e.g. a wash cloth). Once an object has been grasped, stability is maintained by automatically adjusting the force of the grip on the basis of feedback from skin and muscle receptors. If the object is smooth it provides less friction with the skin, and the force of the grip is greater to prevent slip [46].
Table 2.4 Common hand grasps [49–52].
Grasp | Description |
Cylindrical | Whole palmar surface grasps around a cylindrical object, with the thumb flexed and opposed on the opposite side of the object from the fingers (holding a jar or drink bottle) |
Hook | Fingers extended at MCP joints, flexed at IP1 joints. The only functional grasp that doesn’t involve the thumb (lifting a suitcase) |
Lateral(or key grip) | Thumb pad is pressed against the lateral side of the flexed index finger, near the distal IP joint (holding a playing card or a key) |
Locking | Ulnar side of the hand, particularly the little and ring fingers, provide stability for the object being grasped. Middle, ring and little fingers flex over object (holding a knife and fork when eating) |
Oppositional (or subterminal pinch) | Pads of the index finger and thumb are used to grasp, with finger MCP and PIP joint flexion, thumb joints and finger DIP joint flexed (holding a coin) |
Palmar | Fingers hold an object into the palm, all joints flexed, thumb assists (similar to spherical power grasp) |
Pinch (precision or terminal pinch) | Flexion at all index finger joints, thumb MCP joint extension with IP joint flexion so that the tips of the pads are opposing one another (removing a piece of fluff from clothing) |
Power | Fully flexed fingers with thumb flexed and opposed over fingers. Force is applied through fingers into the palm (holding a hammer or a bat) |
Raking | Fingers ‘rake’ the object into the palm and hold it there without thumb involvement (a baby picking up a rattle or sultanas) |
Spherical (or ball grip) | Finger and thumb tips are opposed to hold a small round object, or a larger round object is held against the palm (holding a strawberry or an apple) |
Supporting | Finger MCP flexion and IP joint extension, with either extension of all thumb joints or thumb IP flexed over the object to stabilise it (carrying a plate) |
1Interphalangeal
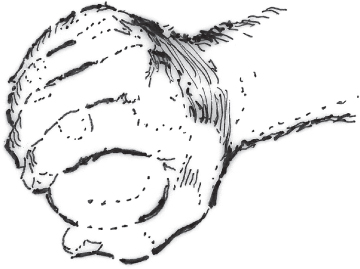
Figure 2.3 Usual position of the hand for function.
2.3 Brain injury and movement dysfunction
The child or adult living with the consequences of brain injury faces many challenges, one of these being limitation to upper limb movement [2]. Movement has been described as “the visible behavior that supports occupation” or daily function [53, p. 236]. However, upper limb movement problems following brain injury often result in limited potential for participating in daily activities. The movement and positioning difficulties that people experience after brain injury occur as the result of changes to many different parts of the movement system, from the brain to the muscles themselves [1].
Clinicians working with people with brain injury have acknowledged that identifying the specific contributors to each person’s movement limitations is often difficult and complex since “every patient is different” [54, p. 19]. Yet despite individual differences, some common upper limb positioning and movement problems are accepted as characteristic of the child or adult with brain injury. Clinically, the following characteristics are regularly seen in differing combinations and to varying degrees:
- Posturing in positions of deformity at rest and/or during active movement
- Compensatory or stereotypical (characteristic, inflexible) movement patterns
- Movement within limited ranges of motion
- Changes in muscle tone at rest and on activity
- Muscle and joint stiffness
- Reduced joint stability and integrity
- Muscle weakness or paralysis
- Perceptual and sensory changes [3, 42, 55].
Although a person’s movement following brain injury may be inefficient and/or ineffective, it nevertheless represents the best effort of his or her movement system given the particular disruption that it has experienced [56]. Observation combined with formal assessment of an individual’s positioning at rest or during movement assists in identifying characteristic problems and relating them to the possible underlying reasons (impairment or pathophysiology) for those problems. Knowledge about the likely causes of impairment allows the clinician to apply specific interventions with a well-reasoned rationale [11, 57, 58]. The following section focuses on the underlying reasons for changes in upper limb positioning and movement following brain injury. Assessment is addressed in Chapters 4 and 5, intervention choices will be introduced in Chapter 2 and discussed in more depth in Chapters 6 to 11.
2.4 Upper motor neuron syndrome
The term upper motor neuron syndrome (UMNS) is used to describe the characteristic changes that become evident in positioning and movement after sustaining a brain injury that affects the cortical motor areas and the descending corticospinal (pyramidal) and/or brainstem (parapyramidal) motor pathways or tracts. UMNs from these pathways extend from the motor areas of the cortex all the way to the end of the spinal cord (see Section 2.1.1 and Table 2.1). Therefore, disruption anywhere along the pathway (cortex, internal capsule, brainstem or spinal cord proximal to the anterior horn cell) leads to presentation of the UMNS. UMN injury interferes with the balance of supraspinal regulation on the alpha motor neurons at the spinal level. Simultaneously, feedback from the somatosensory system (including the muscle spindles, golgi tendon organs, superficial and deep cutaneous receptors) sends excitatory signals to the same spinal level neurons, leading to hyperactive spinal cord reflexes. The result is an overall disturbance in the way that muscles react to stimulation or movement, leading to hypertonia or hypertonicity see Section 2.4.2.9 [11, 40, 56, 59, 60].
The features (or symptoms) of the UMNS that influence a person’s positioning and movement are typically described as either positive (movement excesses, exaggerations or ‘signs of presence’) or negative (movement deficits or ‘signs of absence’), see Table 2.5 [60, 61]. Negative features are usually most easily noticed during the acute phase after an acquired brain injury, when a loss of all spinal reflex activity (called spinal shock) is common, and leads to paresis, flaccidity or loss of muscle tone. Positive features typically emerge more gradually as time progresses post injury (Figure 7.1). When they do appear, they are often readily apparent and may cause underlying negative features to be overlooked [19, 62, 63].
Table 2.5 Positive and negative features of the upper motor neuron syndrome [60, 61].
Upper motor neuron syndrome features | Observed signs and symptoms |
Positive features (excess or exaggeration) |
|
Negative features (deficit or absence) |
|
Adaptive changes |
|
Typically, however, positive and negative features of the UMNS are present in the limb at the same time and together they lead to positioning and/or movement dysfunction that is exacerbated by secondary adaptive (rheologic) changes in the muscles and connective tissues (Table 2.5). The relative contribution of different features differs across individuals, depending on the type and severity of the person’s injury.
Lists of positive and negative features often include slightly different features or terminology, leading to confusion about the underlying causes of the positioning and movement impairments that are observed in the upper limb after brain injury. For example, ‘hypertonicity’ is not usually described as a positive feature in the literature. This increased resistance to movement that is observed clinically includes a neural, reflexive component (usually spasticity, although other tonal disturbances may also be involved) and a non-neural, biomechanical component (arising from muscle and connective tissue stiffness). It can be identified as a positive feature because it often results in excesses of particular undesired joint positions, for example, too much flexion, too much adduction and so on. Another example is ‘learned non-use’ of the affected upper limb which is not usually described as a negative feature on its own. However, it is certainly a ‘performance deficit’ and contributory factors appear to involve reduced somatosensory awareness, movement initiation and learning factors. Therefore, it is included here as a negative feature, see Section 2.4.1.3.
2.4.1 Negative features: performance deficits
Negative features of the UMNS are increasingly viewed as significant factors limiting the achievement of functional independence for people with brain injury (see Table 2.5). The main reasons for the emergence of negative features following brain injury are the reduction in descending, supraspinal influences on motor units and consequent changes in motor unit functions [19, 42]. Negative features include muscle weakness (insufficient muscle activation or paresis), poorly differentiated muscle control [40, 60, 64] and learned non-use.
2.4.1.1 Muscle weakness
Muscle weakness can vary following brain injury, from partial or mild loss of strength (paresis) to severe (plegia) or complete (paralysis or flaccidity) loss of strength [3]. Weakness is a reduced ability to voluntarily generate and sustain sufficient force (or torque) for effective movement or task performance [19]. The consequences of muscle weakness include slowness to develop force, increased fatigability, reduced endurance and an increased sense of effort [65]. Flaccidity occurs when most spinal reflex responses, including stretch reflexes, are depressed, leading to an extreme form of muscle weakness. Flaccidity is usually an immediate and transient response to brain injury, however, when it persists, it is correlated with poorer functional outcome [19]. The importance of muscle strength for upper limb function is indicated by reports that the severity of paresis in the first month following stroke is the strongest predictor of upper limb functional outcomes at six months [66–68], and that voluntary finger extension and shoulder abduction within five days of stroke predict upper limb functional recovery [69, 70]. Wrist and grip strength are described as indicators of upper limb function for children with hemiplegia [71]. Further, overall upper limb strength is reported to directly influence activity measures and, therefore indirectly, to influence functional or participatory outcomes for children with cerebral palsy [72].
Normal muscular force production depends on the number and type of motor units recruited in a contraction, as well as their firing rates (see Section 2.1.2.1). Various physiological contributors to muscle weakness have been proposed following brain injury (Table 2.6). After stroke, motor units are recruited at lower-force thresholds (that is, less effort is required to recruit more motor units) and in a changed pattern. They also fire less rapidly and do not increase their firing rates appropriately in response to movement needs [65, 73–75]. It is possible that the inability to regulate firing rates within optimal ranges leads to greater reliance on over-recruitment in order to control force [40] and to difficulty producing force at shortened muscle lengths [76]. Clinically, over-recruitment of motor units presents as muscle overactivity, and inclusion of more muscles and joints than necessary for particular movements [77]. Difficulty in maintaining a constant force level due to reduced firing rates of agonist motor units may reduce performance of finely controlled movements and fine motor dexterity [18]. In contrast, prolonged firing rates may adversely affect the mechanical properties of associated muscle fibres, leading to fatigue and weakness, both post-stroke and in children with cerebral palsy [19, 78].
Table 2.6 Contributors to agonist muscle weakness after brain injury [19, 65, 74, 76, 78–81].
Contributor | Effect |
Central Nervous System: Reduced descending activation of the spinal-level motor neuron pool |
|
Somatosensory system |
|
Skeletal muscles: Reduced innervation of muscle fibres due to degeneration of motor neuron axons |
|
Selective loss of large motor units due to lack of stimulation (because of degeneration of the descending corticospinal tract at the segmental, spinal cord level) has been identified as an important contributor to muscle weakness [82]. Research has indicated that functioning motor units are halved in the first 6 months following stroke [83, 84] and that reductions are evident as early as 4–30 hours after stroke [85]. In addition, degeneration of type 2 (fast-contracting) motor units combined with an increased number or density of type 1 (slow-contracting) motor units reduces the force-producing capacity of adults with stroke and children with cerebral palsy [18, 65, 82]. Increased fibre density in muscles is an indicator of motor unit reorganisation in response to denervation of the muscle after motor neuron lesion. It develops because intact type 1 motor units send collateral nerve sprouts to previously denervated muscle fibres, leading to an increase in the number (density) of muscle fibres per motor unit in affected areas [82]. Post-stroke, the presence of a fourth type of motor unit characterised by slow contraction and increased fatigability is also reported to have been identified in people with long-standing hemiparesis [18].
In the upper limb, extensor muscles have been reported to be more affected by weakness than flexor muscles [76]. Reduced activity has been noted in shoulder abductor muscles although, in general, distal muscles on the impaired side have been identified as more affected, with greatest weakness found in wrist and finger muscles [86]. Evidence suggests that adults with hemiplegia due to stroke experience impairment of the ipsilateral (non-affected) upper limb in addition to the expected weakness in the contralateral (affected) upper limb [86, 87]. Similarly, children with either hemiplegic or diplegic cerebral palsy have impairments in their apparently non-affected upper limbs in addition to more severe impairment in their affected upper limbs [88]. These outcomes for the less-affected upper limb are proposed to reflect uncrossed fibres in the lateral corticospinal tract for both adults with stroke [89] and children with cerebral palsy [88].
2.4.1.2 Loss of selective muscle control
The severity of the central lesion experienced by a person with brain injury is thought to be reflected in his or her ability to isolate a movement without activating other parts of the limb, with more severe injuries leading to greater impairment in the person’s ability to coordinate limb segments for organised movement [90]. Following brain injury, loss of selective muscle control leads to difficulties with fractionation (isolation or differentiation) of movements, meaning that it is difficult to separate movement in one joint from movement in another joint in a limb. Thus, a person might consistently be observed to use the same inefficient and excessive movement pattern to try and achieve a number of movement goals [40, 42, 90]. For example, the person may habitually flex the elbow and abduct the shoulder while trying to reach forward, but may also use the same pattern when attempting to lift and carry an object.
In the UMNS, poorly differentiated muscle control may present in a variety of ways, including movement synergies, ataxia, dyspraxia or apraxia [40, 64, 75]. Each of these impairments adversely affects voluntary goal-directed movement [60], and this lack of upper limb control is often described functionally as reduced dexterity [11]. Ada and Canning [91] define loss of
“dexterity (motor control) … [as] a loss of coordination of voluntary muscle activity to meet environmental demands, and it is not restricted to manual dexterity … it is a loss of both the spatial and temporal accuracy needed [for] movement” [pp. 95, 97].
In addition to this definition, ‘dexterity’ has also been used to describe the capacity of isolating muscles for discrete movements. It is possible that disrupted transfer of sensorimotor information from the environment may affect selective muscle control and, therefore, both gross and fine motor upper limb dexterity [42].
The use of ‘obligate’ movement synergies or stereotypical patterns is one possible result of the inability to isolate relevant muscles after brain injury [40, 64]. A synergy is defined as “patterned movement of the entire affected limb in response to a stimulus or to voluntary effort” [92, p. 668], and is different to an associated reaction (see Section 2.4.2.8). Muscle synergies occur normally within the intact musculoskeletal system, their purpose being to reduce the degrees-of-freedom needing to be controlled to produce efficient limb use by providing a set of ‘ready solutions’ or ‘movement modules’ [93, 94]. However, in people with brain injury, movement synergies are consistently (inappropriately) used, regardless of the intended end goal of the movement. The precise reasons for such synergies are unclear. It has been suggested that brain lesions might interfere with the structure of the synergies that the CNS typically uses as motor modules for directing movement, therefore causing impaired action [93–95]. Another possibility is that post-injury plasticity promotes connections between the reticulospinal (brainstem) pathway and the corticospinal pathway. The reticulospinal pathway controls gross, multi-joint movement, while the corticospinal pathway controls distal, goal-directed forearm/hand movement. Connections between them may therefore lead to abnormal ‘neural coupling’ of the shoulder/arm and hand joints resulting in upper limb synergies [96]. Further, Roh et al. [95] have proposed that both these possible explanations for movement synergies may occur together, significantly reducing capacity for efficient, goal-directed movement. A number of upper limb synergies commonly observed in children with cerebral palsy and adults with stroke are presented in Table 2.7.
Table 2.7 Common upper limb synergies.
Synergy | Joint positioning |
Brunnstrom’s flexion synergy (stroke) [92] | Scapular elevation/retraction; shoulder abduction/external rotation; elbow flexion; forearm supination |
Brunnstrom’s extension synergy (stroke) [92] | Scapular protraction; shoulder horizontal adduction/internal rotation; elbow extension; forearm pronation |
Abduction/flexion synergy during reach (stroke) [96, 98] | Shoulder abduction; elbow flexion; wrist and finger flexion |
Lower limb extension/upper limb flexion synergy when walking (stroke) [99, 100] | Elbow flexion; finger and thumb flexion |
Adduction/internal rotation synergy during reach (cerebral palsy, spastic hemiplegia) [101] | Shoulder adduction/internal rotation; elbow flexion; forearm pronation; wrist flexion/ulnar deviation; trunk forward flexion |
Abduction/external rotation synergy during reach (cerebral palsy, spastic hemiplegia) [101] | Shoulder abduction/external rotation; elbow flexion; forearm pronation; wrist flexion/ulnar deviation; trunk lateral flexion |
Flexion/over-extension synergy when reaching to grasp (cerebral palsy, spastic hemiplegia) [102] | Wrist flexion; over-extension of fingers |
Thumb-index finger synergy (stroke) [103] | Thumb extension; index finger flexion |
In children with cerebral palsy, ataxia is described as a negative feature of the UMNS. It is defined
“as an inability to generate a normal or expected voluntary movement trajectory that cannot be attributed to weakness or involuntary muscle activity about the affected joints” [64, p. 2162].
Thus, ataxia leads to impairment in joint positioning when moving the limb through space, as well as reduced accuracy due to an inability to activate the correct pattern of muscles for movement. Additional deficits considered to be components of ataxia include dysmetria (inaccurate movement leading to either under- or over-shooting an object), dyssynergia (degeneration of multijoint movements) and dysdiadochokinesia (difficulty performing rhythmic movements) [64]. In adults with stroke, ataxia is described as a loss of movement coordination, and is most apparent during fast, multi-joint movements in chronic stages of stroke [97].
Apraxia is another negative feature of the UMNS described as affecting the movement of children with cerebral palsy. It is defined as
“impairment of the ability to accomplish previously learned and performed complex motor actions that is not explained by ataxia, reduced selective motor control, weakness, or involuntary motor activity” [64, p. 2163].
Apraxia is distinguished by its presence during task-related activity, while ataxia is apparent during simpler, non-purposeful movements.
2.4.1.3 Learned non-use
Although not specifically described as a negative features of the UMNS, learned non-use of the hemiplegic upper limb following brain injury has become recognised as a significant contributor to decreased upper limb function [62]. Reduced use of the affected upper limb is proposed as being ‘learned’ as a response to the increased effort needed to move the limb due to muscle weakness combined with the inability to selectively control the amount and timing of movement needed to achieve intended tasks. Since movement is so effortful and functional outcomes so poor, people with hemiplegia are proposed as being less motivated to use the affected arm, resulting in learned non-use of that arm and hand [104]. In effect, an ongoing cycle of paresis/weakness-disuse-paresis/weakness is set up which can lead to tissue shortening and the development of contracture [18].
More recently, factors in addition to learning and motivation have been suggested as influencing non-use of the hemiplegic upper limb. Van der Lee [105] suggests that sensory disorders may be the most substantial contributor to learned non-use. This assertion is based on studies in which monkeys with deafferentation had little motor impairment but significant sensory impairment. In addition, Wolf [106] has used imaging studies with stroke-affected and healthy participants to propose that structural changes to areas of the brain involved in movement initiation (cingulate motor and pre-frontal areas) may cause reduced motivation to move prior to any learning effects.
2.4.2 Positive features: performance excesses
Positive features of the UMNS include excesses or exaggerations of reflex activity, muscle activity or movement (see Table 2.5) [107]. Over time, views have changed on whether intervention ought to focus on positive or negative features of the UMNS, particularly spasticity (see Section 2.4.2.6). However, the degree of influence of positive and negative features on a person’s movement is dependent on the type and extent of brain injury. For example, the limb of a person with flaccid hemiplegia due to stroke or cerebral palsy is more affected by widespread loss of muscle strength (a negative feature), whereas the person who has experienced a high brainstem injury is likely to experience significant spasticity (a positive feature). Usually, though, it is the interaction of both negative and positive features that adversely impact a person’s movement and, therefore, the person with brain injury is best served by a clinician who is able to effectively discern the relative contribution of both positive and negative features to movement impairment (see Section 4.3.2).
Positive features of the UMNS typically emerge in people with stroke or traumatic brain injury following a period of reduced muscle activity (spinal shock or flaccidity). Positive features include hyperreactive reflexes at rest (for example, stretch reflexes, deep tendon reflexes, the Babinski or extensor plantar response, flexor and extensor spasms) and muscle overactivity at rest or during movement (for example, co-contraction, dystonia and associated reactions). The mechanisms underlying positive features are not clearly understood, although loss of supraspinal inhibitory control is recognised as being responsible for the majority of the symptoms observed clinically, together with structural and/or functional reorganisation of related spinal mechanisms (Table 2.8) [11, 40, 58, 63]. The underlying neural mechanisms that result in the observed positive features of the UMNS are presented in more detail in Table 2.8. Hypertonicity is not included in this table as it arises from a combination of neural and non-neural mechanisms and is addressed further in Section 2.4.2.9.
Table 2.8 Neural mechanisms underlying positive features [11,19,40,58,63].
Neural mechanism | Observed positive features |
| |
|
|
|
|
|
|
|
|
a Tonic stretch reflexes develop sustained tension in affected muscles in response to longer passive muscle stretch.
b Phasic stretch reflexes are transient and are stimulated by brief, sudden changes in stretch, see Box 2.4.
c The clasp-knife response is another symptom of the tonic stretch reflex underlying spasticity.
d Brainstem efferent (motor) pathways include the rubrospinal, tectospinal, reticulospinal and vestibulospinal tracts, see Box 2.5.
2.4.2.1 Hyperactive tonic and phasic reflexes
Exaggerated, hyperactive or hyperexcitable spinal reflexes, evident either while the person is at rest or during movement, are responsible for most of the positive features of the UMNS that are observed clinically (Table 2.8). Spinal reflexes become hyperactive due to disruption of the regulating influences of the descending pathways involved in motor control, that is, the closely-associated corticospinal (pyramidal) and brainstem (parapyramidal) pathways. These pathways control stretch, flexor and extensor reflexes by modulating (adjusting) signals to the motor neurons in the spinal cord on the basis of sensory feedback from the periphery [11, 77]. It is most likely that a reduced threshold is the reason that the motor neuron is hyperactive. A reduced threshold means that, functionally, a smaller stretch at a slower than usual velocity results in a larger that usual response in the tested muscle. Studies suggest that, following UMN injury, motor neurons are constantly close to their threshold for excitation and therefore require little further input in order to fire [11, 61].
The stretch (or myotatic) reflex is the simplest example of a monosynaptic, short-latency, segmental spinal reflex. It comprises a receptor (muscle spindle), a sensory (Ia primary or afferent) neuron which takes information to the spinal cord, and a motor (alpha [α] or efferent) neuron that activates the fibres in the same muscle as the receptor (Figure 2.4). The stretch reflex is a proprioceptive reflex that is present in all muscles, both flexor and extensor, and is described as either phasic or tonic. It is triggered mechanically, by a rapid (phasic) stretch of the muscle, usually by moving the joint quickly through its range of motion or by tapping the relevant tendon. The stretch reflex that occurs following a tendon tap is known as a deep tendon reflex (DTR), a tendon reflex or a T-reflex. The tonic stretch reflex arises from a sustained muscle stretch and is the cause of spasticity (further discussed in Section 2.4.2.6) [33, 111]. See Box 2.4 for further information about reflexes.
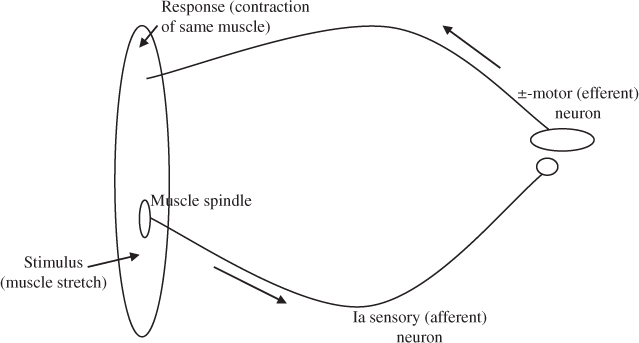
Figure 2.4 Stretch (myotatic) reflex (adapted from [33]).
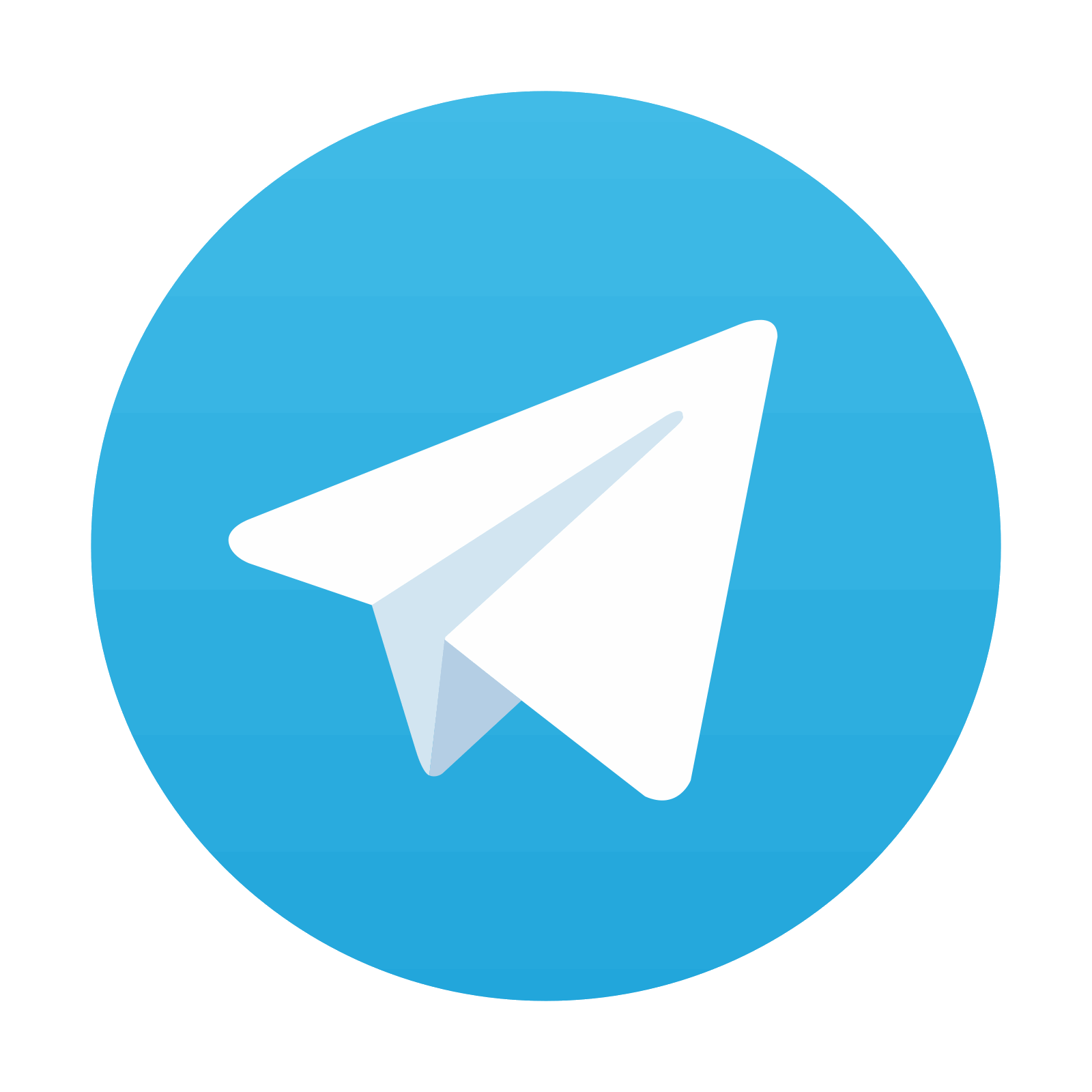