1 Anatomy and physiology of the cerebrospinal fluid system
Adult Hydrocephalus, ed. Daniele Rigamonti. Published by Cambridge University Press. © Cambridge University Press 2014.
Introduction
Water distribution of the brain
In the average human, the total body water is about 60% of the body weight [1]. The brain is highly sensitive to the amount and distribution of water in the intracranial space. The intracranial space enclosing the brain has a capacity of about 1600–1700 ml. About 100–150 ml of this capacity is occupied by cerebrospinal fluid (CSF) [1]. Other components in the intracranial cavity include blood (100–150 ml). The remaining volume is comprised of brain parenchyma and intracellular and interstitial spaces. The brain parenchyma, CSF, and blood all interact with one another in a pressure–volume relationship known as the Monro–Kellie doctrine. According to the Monro–Kellie doctrine, the volume inside the cranium is a fixed volume and the intracranial components are in equilibrium such that an increase in one component (i.e. brain parenchyma) must lead to a compensatory decrease in another component. Since the brain parenchyma is minimally compressible, the primary buffers for increased intracranial volumes are CSF and to a lesser extent blood. The total intracranial and extracranial volume of CSF is divided between the ventricular system (35 ml, 25%), the spinal canal (30–70 ml, 20–50%), and the cranial subarachnoid space (35–75 ml, 25–55%) [2,3]. Cerebrospinal fluid is formed at a rate of approximately 500 ml each day. This volume is three to four times as much as the total volume in the entire CSF system and reflects that CSF is constantly being replaced [1].
CSF function
CSF provides physical protection for the brain and spinal cord through buoyancy and thus by reducing the active weight of the nervous structures. Immersed in CSF, the active weight of the brain is reduced from 1500 g to as few as 50 g thus reducing tension on the nerve roots and vessels and the risk of injury in case of trauma [4]. More importantly, CSF functions as a reservoir to get rid of metabolites and toxins from the brain. It also plays a role in homeostatic hormonal signaling, chemical buffering, circulations of nutrients, and neurodevelopment [4–7].
CSF composition
Under normal conditions, CSF is a clear and colorless fluid with a density ranging from 1.003 to 1.008 g/cm3. Compared to plasma, CSF has a lower concentration of glucose, protein, and potassium while having a higher concentration of chloride. The CSF protein concentration in adult mammals is 2–3 orders of magnitude lower than plasma, reflecting the degree of impermeability of the blood–CSF barrier to macromolecules [8]. Nevertheless, a great amount of research is being done on protein composition and the role it may play in disease states. β2-transferrin is a special iron-binding glycoprotein only found in CSF, perilymph, and ocular fluids that can be used to determine whether watery discharge from a patient is CSF [9,10].
Anatomy of the CSF system
The CSF system is a multi-compartmental large-cavity fluid system encompassing the ventricles, cisterns, and subarachnoid space (SAS), all serially linked with one another. The two lateral ventricles drain into the third ventricle through the foramina of Monro. The third ventricle subsequently drains into the fourth ventricle through the narrowest portion of the ventricular system called the cerebral aqueduct (of Sylvius). From there CSF enters the subarachnoid cisterns around the brain through the foramen of Magendie and the foramina of Luschka.
Although CSF is contained within the walls of the ventricular system, ventricular CSF is functionally interactive with the ependymal walls of the ventricles as well as the underlying brain capillary surfaces [11]. Bulk flow and diffusion promote water and solute distribution in and out of the brain, depending upon prevailing regional concentration gradients and hydrostatic pressure gradients.
Anatomy of the lateral ventricles
The two lateral ventricles are mirror images of one another, separated by the septum pellucidum and septal nuclei. The inferolateral portion of the frontal horn, body, and atrium of the lateral ventricles comes in contact with the caudate and then the thalamus more posteriorly. The portions of the lateral ventricles are bounded superiorly by the corpus callosum and forceps. Laterally, the lateral ventricles are bordered by the cerebral white matter between the caudate and thalamus below and the corpus callosum above [12].
The temporal horn of the lateral ventricles is surrounded by the hippocampus medially, the parahippocampal white matter inferiorly, and the temporal lobe white matter laterally and superiorly. The tail of the caudate nucleus also runs along the roof of the temporal horns. The amygdala also abuts the medial portion near the temporal horns.
The occipital horn of the lateral ventricles is completely encompassed by the white matter of the occipital lobe except when the calcarine fissure encroaches near the calcar avis.
Anatomy of the foramina of Monro
The anterior and medial border of the foramen of Monro is the fornix, whereas the posterior and lateral circumference of the foramen of Monro is bounded by the thalamus and choroid plexus. The choroid plexus courses anteriorly along the choroidal fissure (a cleft between the fornix medially, and the thalamus laterally) to reach the inferolateral aspect of the foramen of Monro. The thalamostriate vein runs in a sulcus that separates the caudate nucleus and thalamus. The confluence of the thalamostriate vein and the septal vein gives origin to the internal cerebral vein. The point of confluence is the venous angle – an angiographic landmark for the foramen of Monro on lateral view angiogram.
Anatomy of the third ventricle
The midline, normally slit-like third ventricle is bounded laterally by the thalami. On the anteroinferior border there is the hypothalamus. The interthalamic adhesion (massa intermedia) traverses the third ventricle. The floor of the third ventricle is comprised of the tuber cinereum anteriorly whereas the posterior portion is comprised of the mammillary bodies and the rostral portion of the midbrain. The lamina terminalis forms the anterior wall and contains the anterior commissure superiorly and the optic chiasm inferiorly. The optic recess is found on the anterior aspect of the floor of the third ventricle just above the optic chiasm. More posteriorly is the infundibular recess. The roof of the third ventricle is comprised of the tela choroidea, which contains the internal cerebral veins and the posteromedial choroidal arteries and is a continuation of the choroid plexus that runs across the lateral ventricles into the foramina of Monro and then along the roof of the third ventricle. The internal cerebral veins drain the choroid plexuses and join to form the great cerebral vein (of Galen).
Cerebral aqueduct
The cerebral aqueduct connects the third and fourth ventricles and is on average 15 mm in length in adults with a diameter varying from 0.5 to 2.8 mm along its course. It is surrounded by the periaqueductal gray matter of the midbrain. Anterior to the cerebral aqueduct lie the tegmental midbrain structures including the oculomotor and trochlear nuclei. Posterior to the cerebral aqueduct is the midbrain tectum comprised of the superior and inferior colliculi, which contribute to the strictures in the cerebral aqueduct [13].
Anatomy of the fourth ventricle
The fourth ventricle is located behind the pons (rostral half) and the medulla (caudal half). It is bounded laterally by the superior, middle, and inferior cerebellar peduncles. The roof of the fourth ventricle is bounded by the vermis of the cerebellum (superior half) and the fastigium (inferior half). The caudal portion of the roof of the fourth ventricle opens into the cisterna magna via the foramen of Magendie medially and the foramina of Luschka laterally. The inferior apex of the fourth ventricle is surrounded by the obex and is continuous with the central canal of the spinal cord.
Overview of the cisterns
The cisterns are CSF spaces situated between the brain and skull separated from each other by trabeculated porous walls of arachnoid with various size openings. They typically contain traversing arteries, veins, or nerves. These cisterns are bounded by septations, which may become inflamed and fibrotic thus limiting circulation of CSF [12]. There is increasing research now suggesting that this pathology may contribute to some cases of communicating hydrocephalus. CSF empties into the cistern magna via the medial foramen of Magendie and the lateral foramina of Luschka (Figure 1.1). The CSF then circulates in the subarachnoid cisterns surrounding the brainstem (i.e. prepontine, interpeduncular, and ambient cisterns) to reach the cerebral convexities. Ultimately, CSF will diffuse passively into the venous sinuses via the arachnoid villi.
Figure 1.1 Overview of the ventricular system and cisterns. CSF is produced and flows from the lateral ventricles into the third and then fourth ventricles. From the fourth ventricle, CSF empties into the cisterns of the skull base, which will then flow into CSF cisterns of the cerebral convexities.
Cerebral convexity CSF spaces
The cerebral convexity CSF spaces are located over the cerebral cortex and within sulci as well as between the dura. Much of the CSF reabsorption will occur at the arachnoid granulations of the dural sinuses. The cerebral convexity CSF spaces are large in fetuses and become progressively smaller towards childhood and adolescence. However, they enlarge again in old age, most prominently over the parietal lobes.
Spinal CSF anatomy
The CSF spaces of the spine extend from the foramen magnum down to the filum terminale surrounding the spinal cord and the nerve roots. The CSF spaces extend laterally from the vertebral foramina to the nerve sheaths of the spinal sensory ganglions. These nerve sheath diverticula have mechanical purposes (e.g. sliding motion to the spinal nerves and elasticity to the meninges) as well as serving as sites for CSF reabsorption.
CSF production
About 500 ml of CSF is produced each day in the average human adult. The choroid plexus is responsible for 60–70% of CSF production. Another 30–40% comes from the interstitial fluid exudated from the pia from vessels as well as metabolism. CSF production is to a degree dependent on blood perfusion. In instances of increased intracranial pressure (ICP) with subsequent decreased cerebral perfusion, there will be a decrease in CSF production.
Choroid plexus
Structure
The choroid plexus is located in the roof of the temporal horns of the lateral ventricle and the roof of the third and fourth ventricles and rests on the floor of the frontal horns and atriums of the lateral ventricles. It is comprised of vascular pial stroma covered by a choroidal epithelium (Figure 1.2). The choroid plexus contains a vascular endothelium that is fenestrated, unlike most vessels of the brain [4]. The zona occludens that connect the choroidal endothelial cells still permit most small hydrophilic molecules to pass [3]. The tight junctions present on the apical surface of choroid plexus epithelial cells, however, provide for tightly sealed junctions in contrast to the ventricular ependyma [4].
Figure 1.2 The choroid plexus has a “hobnail” appearance. It consists of a layer of cuboidal epithelial cells that surround an inner core comprised of connective tissue, pia mater, and capillaries.
CSF secretion
The primary function of the choroid plexus is the secretion of CSF, which is accomplished by water ultrafiltrate following an osmotic gradient produced by ions such as Na+, Cl–, and HCO3–. Active transport with ATP hydrolysis occurs to create a unidirectional flux of these ions across the choroid plexus epithelial layer, in turn driving osmotic transcellular movement of water [14,15]. The aquaporin-1 channel located on the CSF-facing apical surface of the choroid epithelial cells allows for water movement [16]. Aquaporin-1 inhibition has been proposed as a method to treat hydrocephalus; however, studies with aquaporin-1 knockout mice still produce a normal amount of CSF, suggesting other mechanisms of water transport [17]. Nevertheless, CSF production is known to be reduced by acetazolamide, diuretics, changes in CSF osmolality, steroids, and low temperatures [18].
Other functions
In addition to its CSF secretory function, the choroidal epithelium synthesizes a large number of bioactive peptides [6]. These bioactive peptides include insulin-like growth factor II, transforming growth factor beta, fibroblast growth factor, human growth factor, arginine vasopressin, and some bone morphogenetic proteins [11]. These CSF peptides can act on brain structures such as the hypothalamus or chemosensitive structures of the medulla oblongata. They can also act on the choroid plexus itself in an autocrine fashion [11].
The choroid plexus may also be involved in eliminating endogenous waste and foreign substances from the CSF, thus cleansing the CSF and adjusting CSF and brain extracellular fluid [11,19,20]. On the apical surface of choroid plexus epithelial cells have been found peptide transporters, multidrug resistance-associated proteins, organic anion-transporting polypeptides, and organic ion transporters [11,20]. Furthermore, the continual turnover of CSF is a mechanism to rid the brain of metabolic wastes and also maintain optimal pH.
Interstitial fluid contribution to CSF production
Anywhere from 10% to 30% (but possibly as much as 60%) of CSF fluid is thought to arise from bulk flow of interstitial fluid that derives from transport across blood vessels and from endogenous metabolism that produces water byproducts [4,21]. One large contributor to this volume of fluid is oxidative metabolism of glucose leading to water production, which can produce as much as 12% of net volume of CSF.
CSF flow
CSF flows via net bulk flow from the lateral ventricles through the foramen of Monro into the third ventricle, then on into the fourth ventricle via the cerebral aqueduct. Ultimately, CSF empties out of the fourth ventricle via the midline foramen of Magendie and the lateral foramina of Luschka into the subarachnoid cisterns (there is also a minor amount of CSF that is thought to travel through the spinal central canal straight from the fourth ventricle). Once in the subarachnoid space, the CSF travels over the cortical convexity and skull base, and to a lesser extent into the spinal subarachnoid space until it is ultimately reabsorbed at the dural venous sinuses and around the neural sheaths [22].
Cellular composition of the ventricles
CSF circulation is influenced by the cells, junctional barriers, and membranes lining the ventricular and subarachnoid spaces. In addition to the choroid plexus, the ventricles and the central canal are lined by a continuous single layer of cuboidal and columnar ciliated epithelial cells called ependymal cells [23]. The zona adherens and the numerous gap junctions between these cells allow them to act as a barrier to water movement, although fluid still may permeate into the brain parenchyma. The cilia are believed to possibly contribute to the direction and quantity of CSF flow. Scattered in the layer of ependymal cells are specialized cells, called tanycytes, which have radially directed basal processes that extend into the neuropil where they come in contact with blood vessels. The tanycytes of the third ventricle are believed to help regulate neuroendocrine function as well as guiding functions for hypothalamic axons, and transport between ventricle and blood vessels of the portal system [24,25]. Directly beneath the cells of the ependyma is a basement membrane that forms labyrinths connecting the basement membrane to the perivascular basement membranes of the subependymal capillaries and veins. These glycoprotein and glycolipid basement membranes hold fluid by swelling [26].
CSF flow through the ventricles
CSF is thought to travel through the ventricles in a pulsatile manner with each cardiac systole and respiratory inhalation. There are data from human radiographic imaging studies as well as animal studies showing that cardiac pulsation plays a dominant role in CSF flow [27,28]. Animal data suggest that pressures in the subarachnoid space are also influenced by respiratory function to a great degree [29,30]. Coughing or Valsalva maneuver will cause CSF to flow cranially and then caudally. The cilia of the ependyma most likely play a lesser role in CSF movement; however, there are some animal data suggesting that cilia function is necessary for regulating ion transport and CSF production, as well as for CSF flow through the ventricles [31].
CSF flow through the cranial subarachnoid cisterns and Virchow–Robin spaces
Outside of the ventricles, the intracranial CSF exists within the subarachnoid space between the pia and arachnoid mater. The arachnoid cell layer contributes to the blood–brain barrier and is composed of cells, cell junctions, and an internal basement membrane [32]. The arachnoid consists of an outer portion, the arachnoid barrier cell layer, and an inner part, the arachnoid trabeculae which span the subarachnoid space [33]. Arachnoid barrier cells are closely packed and joined by many cell junctions [33]. Arachnoid trabecular cells comprise the arachnoid trabeculae that span the subarachnoid space, have extracellular collagen associated with their flattened processes, and form cisternal structures of variable shapes and sizes [33]. Nerves and vessels traverse these cisterns. Under normal conditions, CSF flows through the cisterns. Obstruction of CSF flow through the cisterns may explain the development of communicating hydrocephalus in some conditions such as post-meningitic or hemorrhagic hydrocephalus [34].
Another location that has important implications in cerebral fluid movement is the perivascular Virchow–Robin spaces found between the blood vessels and the brain and spine (Figure 1.3). Virchow–Robin spaces are continuous with the subpial and subarachnoid spaces and thus provide a pathway for fluid flow from the subarachnoid space to the brain and spinal cord [35]. This perivascular flow of CSF has been shown in animal models [36,37]. The pulsation of arteries has been suggested as the driving force for perivascular flow [27].
Figure 1.3 Virchow–Robin spaces are fluid-filled spaces surrounding perforating arteries and veins coming into and out of the brain parenchyma, respectively. They are found in the subarachnoid and subpial spaces.
CSF flow through the spinal central canal and subarachnoid space
The central canal plays little to no part in CSF flow. Human cadaver studies have demonstrated central canal stenosis or occlusion in 75–97% of people after the third decade of life [38,39].
Magnetic resonance imaging has suggested that spinal subarachnoid CSF is also greatly influenced by the cardiac cycle. Cardiac systole initiates an anterior and caudal spinal cord movement, followed by caudal CSF flow and then cranial flow during diastole [28,40].
Other possible routes of CSF circulation
Recent advances in molecular biology and ionic physiology provided a different prism for the understanding of biological fluid turnover and homeostasis in biological membranes. The discovery of aquaporins, by Peter Agre, solved a longstanding riddle related to water homeostasis and movement in biology and led to a Nobel Prize in 2003 [41]. Aquaporins, later on, were identified as the most important regulators of water movement and homeostasis in various organisms from bacteria, to plants, to mammals [42]. Interestingly, aquaporin-1 (AQP1) and aquaporin-4 (AQP4) can be identified in abundance in the central nervous system. AQP1 is predominantly identified at the apical (CSF side) of the choroid plexus and the ependyma while AQP4 is found mainly at the brain cortical surface, at the glia limitans layer just below the pia matter, and also can be identified at the astrocytic endfeet, at the level of the blood–brain-barrier (BBB) [42, 43] (Figures 1.4 and 1.5). The presence of aquaporins at anatomical sites related to CSF production or outflow or sites where the brain interstitial fluid (ISF) meets the circulation (BBB) created a strong debate concerning their role in CSF turnover at these sites. Most evidence describing their role is obtained indirectly from studies related to hydrocephalus or AQP knockout mice [44]. In AQP1 knockout (KO) mice, ICP is lower and CSF production is lower too, although a systemic effect at the kidneys was also observed. This observation suggested a role for AQP1 in CSF production or turnover at the AQP1-rich sites such as the choroid plexus and the ependyma [45]. In AQP4 KO mice, kaolin-induced hydrocephalus, ICP, and brain water content were significantly worse than in the controls. These findings suggested that AQP4 could be a significant water channel related to the ISF-CSF drainage in extreme conditions and possibly the sites of drainage could be the AQP4-rich sites such as the BBB and the glia limitans at the subpial zone [46].
Figure 1.4 AQP4 can be identified at the astrocytic endfeet, at the level of the blood–brain barrier and plays a significant role in the movement of water into and out of the brain.
Figure 1.5 AQP4 is found mainly at the brain cortical surface, at the glia limitans layer just below the pia matter.
In the field of biological fluid turnover, especially in preformed spaces lined by biological membranes, water channels work in perfect harmony with ion channels to induce micro-osmotic gradients that create water flux. Ionic flux especially for Na+ and Cl– is translated to paired water flux and this process is well described as “solute-coupled” transport [47–49]. A common example related to CSF turnover is the additive effect of the ion channels at the apical side of the choroid plexus, governed mainly by the effect of the sodium-potassium-ATPase pump that drives sodium into the CSF side, paired with the effect of AQP1 that drives water from the basal site (bloodstream) through the choroid plexus cell to its apical site to the ventricle [50]. Ouabain, a specific sodium-potassium-ATPase inhibitor, can reduce CSF production by 70% [51,52], suggesting that the inhibition of “solute-coupled” transport is an important regulator of CSF turnover. The sodium-potassium-ATPase pump is the cornerstone of “solute-coupled” transport in epithelia [50,51,53,54]. It seems though that there are potentially more sites where “solute-coupled” transport related to CSF turnover occurs. In a seminal paper by Milhorat, CSF turnover was studied by observing the movement of 24Na. Milhorat tried to identify differences between normal monkeys and monkeys that have undergone choroid plexectomy. Interestingly, in plexectomized monkeys, CSF production was reduced by 40%, while 24Na, as an indirect water or CSF marker, was accumulated at brain sites around the ventricles and at sites bordering the subarachnoid space [55]. Thus, a potential avenue for CSF or water movement should also exist at the ventricular ependymal and the subarachnoid space. AQPs, such as AQP1 and AQP4, exist at these brain–CSF interfaces (Figure 1.5). In order to have a “solute-coupled” transport of water, polarized ion channels, and especially the presence of sodium-potassium-ATPAse, are needed at these interfaces. Filippidis et al. identified with membrane electrophysiology that the subarachnoid space behaves as a “leaky epithelium,” an epithelium with high ionic movement and properties similar to mesothelial tissues with an essential role in fluid turnover such as the pleura, omentum, and pericardium [49]. Filippidis et al. also identified a polarized expression of sodium-potassium-ATPase at the subarachnoid space, which could be blocked with ouabain [56], suggesting that at the brain surface–glia limitans–subarachnoid space interface, water or CSF movement could potentially occur by the paired actions of the sodium-potassium-ATPase pump and aquaporins.
Cellular physiology and functional anatomy of the CSF spaces should be studied together to provide a holistic approach to the understanding of CSF physiology. It is coming to be understood that solute-coupled transport and bulk flow are the main forces governing the behavior of CSF flow in the central nervous system, both important at the micro and macro world of CSF production and outflow.
CSF absorption
The classic theory has been that the arachnoid granulations draining into the dural sinuses were the only site of CSF reabsorption but recent data are now suggesting that the mechanism of CSF reabsorption may be more complex than initially thought. There may be other pathways of CSF reabsorption such as the ependymal layer of the ventricular system, endothelial layer of the brain parenchyma, and the lymphatics [57–59].
Arachnoid granulations
Arachnoid villi consist of invaginations of arachnoid into the venous space called arachnoid granulations [60] (Figure 1.6). They are present in the cranial venous sinuses, and to a lesser extent in the venous angles in spinal nerve roots [22]. Arachnoid granulations are dilations of the subarachnoid space within a villus. Arachnoid granulations are more commonly found in adults over 18 years of age, while arachnoid villi have been found in younger infants [61]. Cords of arachnoid membrane penetrate the dura around a draining vein and become a solid mass covered by arachnoid cells. CSF drains into the arachnoid villi from the neck of the villus, which is in contact with the subarachnoid space through dural defects allowing CSF to circulate into the arachnoid villi from the cranial subarachnoid space and ultimately into the venous sinus. CSF flow into the venous sinuses occurs passively via a pressure gradient [22]. Electron microscopy studies have shown that giant vacuoles form that communicate with both the subarachnoid and luminal parts of the cell simultaneously [60]. The greater the pressure gradient, the more vacuoles form.
Figure 1.6 Arachnoid granulations consist of invaginations of arachnoid into the venous space. CSF from the subarachnoid space drains into the arachnoid villi and ultimately into the venous sinus.
Lymphatic drainage
Although lymphatics are not found within the central nervous system, there are experimental data suggesting connections between the brain tissue and cervical lymphatics [62]. One of the most prominent possible routes of lymphatic drainage is via the olfactory nerve rootlets at the cribriform plate with direct communication with lymphatic vessels [63,64]. In fact, some animal studies have suggested as much as 50% of CSF exits the cranium at the cribriform plate in various animal species [65,66]. Injection of various tracers (i.e. Microfil, ink tracers, labeled ink) into the brains of experimental animals or human cadavers had led to accumulation of tracer in the perivascular spaces, and eventually in the cervical and spinal lymph nodes, reaching a peak in 4 to 15 hours [63,67]. Obstruction of the lymphatics from the head in animal models resulted in elevated intracranial pressures [68].
The spinal lymphatic routes of CSF drainage have been proposed as another lymphatic drainage route. In sheep as much as 25% of total CSF drainage can occur via this route. Radionucleotide testing in healthy human volunteers demonstrated that 0.11–0.23 ml/min of CSF could be absorbed from spinal routes, and more during exercise [69]. It has been hypothesized that CSF drainage from the spine occurs at spinal arachnoid granulations as well as at where the nerve sheaths merge with the perineurium at the arachnoid angle. CSF drainage may also occur as a result of direct CSF movement across dura into the epidural space into spinal lymphatic vessels.
Windkessel effect on CSF drainage
Our understanding of the mechanisms related to the absorption of intracranial arterial blood flow energy and how they relate to the absorption of CSF is continuing to evolve. The problem with relying on the Monro–Kellie doctrine is that it cannot explain CSF circulation and absorption in relation to cardiac function, which is why there has been interest in the Windkessel effect on CSF. The Windkessel effect is the energy transfer resultant from arterial blood flow energy formed by cardiac action that is transferred to the intracranial space as arterial blood flow, brain pulsation, and CSF pulsation and bulk flow. Arterial distension occurs during the systolic phase of blood pressure while arterial recoiling occurs during the diastolic phase of blood pressure. This provides for constant blood flow to arterioles and capillaries as a result of energy transferred from the force of arterial distension during the systolic phase to the recoil of the diastolic phase. A portion of this energy is also transferred to the brain in the form of brain pulsation and to the CSF in the form of CSF pulsation and bulk flow. The remaining energy finally dissipates as CSF passes through the arachnoid villi and exits via the venous system via a pressure gradient. The pulsatile flow kinetic energy of CSF is more than 100-fold greater than the bulk flow kinetic energy of CSF and would thus seem appropriate as the source of energy dissipation from arterial blood flow energy. It has been proposed that the CSF pulsation energy dissipates while maintaining a low intracranial pressure by the summation of a diverse array of CSF flow vectors that offset each other in direction and time [70]. This dispersion of CSF pulsation energy has been demonstrated radiographically by the diffuse, random mixing of CSF flow [71–73]. The dissipation of arterial blood flow energy by the CSF pulsation energy provides for the maintenance of a low ICP and subsequently high cerebral blood flow, which is the difference between mean arterial pressure and ICP. The Windkessel effect on CSF may explain why lateral ventricular dilation may occur in communicating hydrocephalus without changes in the mean ICP [70,74].
Conclusion
There is still a great amount that needs to be understood in regard to the dynamics between CSF production and absorption. Further understanding of the physiology and anatomy of the cerebrospinal fluid system will enable scientists and clinicians to develop better ways to approach and treat hydrocephalus.
References









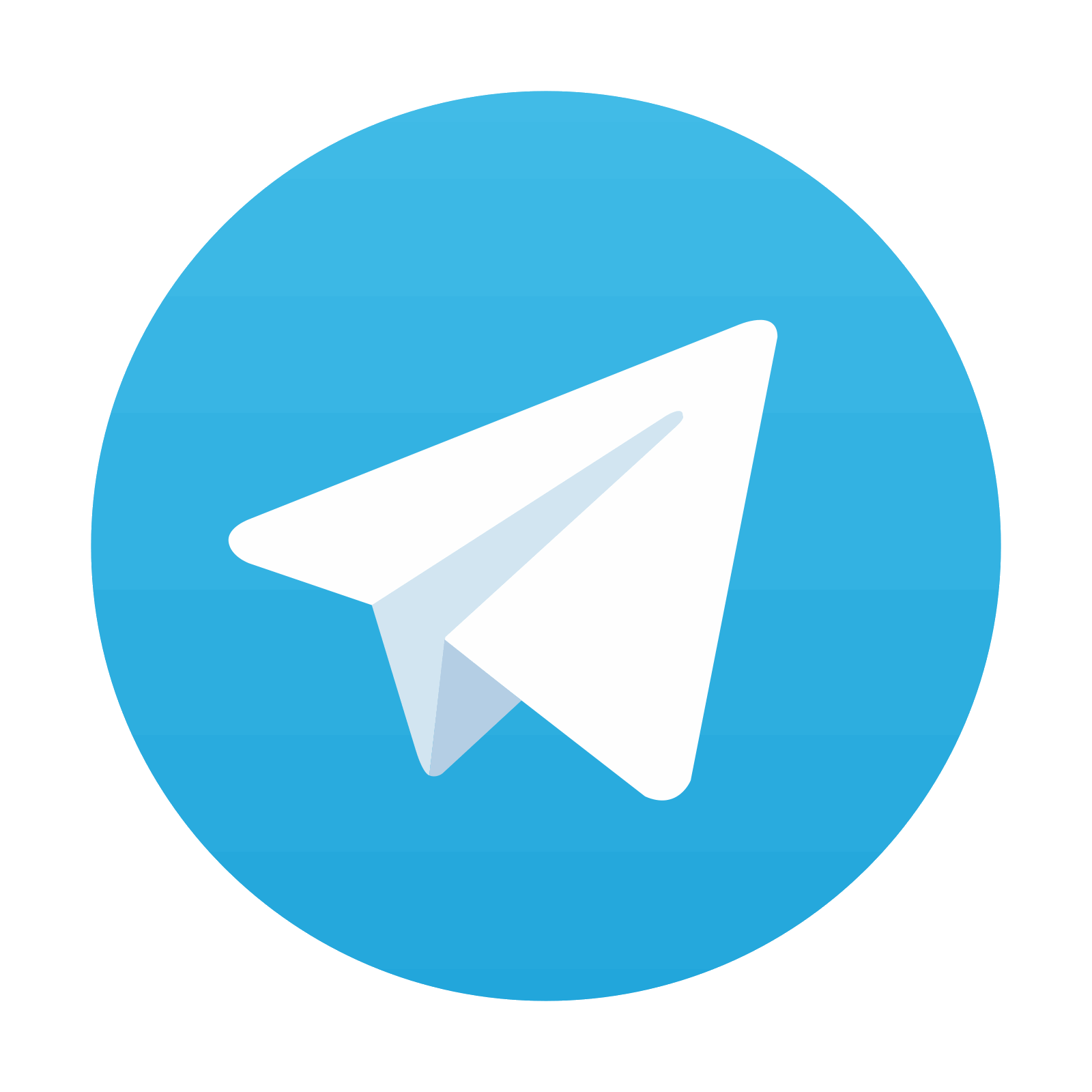
Stay updated, free articles. Join our Telegram channel
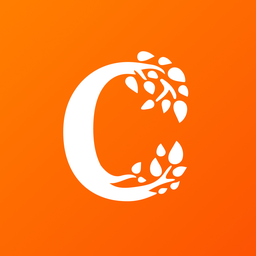
Full access? Get Clinical Tree
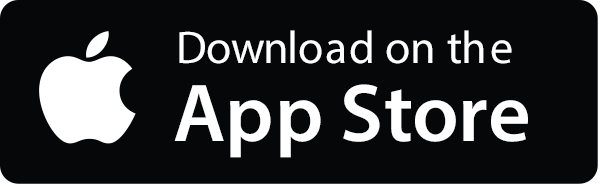
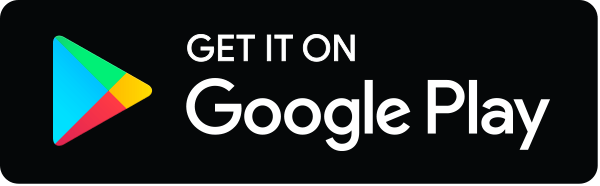
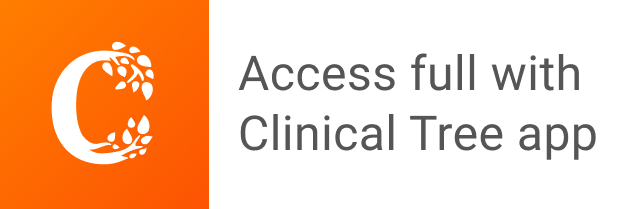