10 Communicating Hydrocephalus

10.1 Introduction
Communicating (nonobstructive) hydrocephalus is a relatively common source of morbidity and mortality in patients with disorders of the central nervous system (CNS) and may arise: (1) congenitally; (2) as a result of precipitating factors, including intracranial hemorrhage (e.g., subarachnoid or intraventricular), CNS infections, leptomeningeal neoplastic spread, trauma, spinal cord tumors,1 radiotherapy,2 or certain drugs3; (3) or for reasons that are unclear (e.g., idiopathic normal pressure hydrocephalus [iNPH]).
10.2 Pathophysiology
The pathophysiology of communicating hydrocephalus is a matter of much debate, and no identifiable consensus currently exists.4,5 The development of communicating hydrocephalus appears to be characterized by derangements of multiple intracranial physiologic variables, including cerebrospinal fluid (CSF) flow dynamics, microvascular pulsatility, and compliance of the subarachnoid space and vasculature, among others. A brief description of the prevailing theories follows.
The conventional “bulk flow” theory of CSF flow dynamics asserts that CSF is produced in a certain location and absorbed in another location, and that CSF flow within the ventricles and subarachnoid space occurs due to small differences in pressure between the sites of CSF production and absorption.6 According to this theory, obstructive hydrocephalus arises due to impaired flow between the ventricular system and subarachnoid space, while communicating hydrocephalus arises from impaired absorption of CSF, trapped cisterns, and/or an immature subarachnoid space.
Early experimental animal studies suggested that the primary site of CSF absorption lay in the pacchionian granulations, through which CSF is absorbed via a hydrostatic and osmotic pressure gradient into the dural venous sinuses and nerve roots.7 Scarring of the arachnoid villi due to insidious inflammatory responses to infection, hemorrhage, trauma, or neoplastic spread were proposed to result in impaired absorption in these arachnoid villi and subsequent development of communicating hydrocephalus.8,9,10
Other authors have rejected the pacchionian granulations as the primary site of CSF absorption, instead asserting that the primary site for CSF absorption is through the choroid plexus and fenestrated venous capillary network existing within the brain parenchyma and subarachnoid space, a theory initially proposed by Dandy,11 and now supported by a growing body of literature.12 In addition, a substantial contribution to CSF production is now known to take place outside of the choroid plexus.13,14 Experimental evidence exists that CSF flow, rather than being driven by differential pressure between the choroid plexus and pacchionian granulations as described in the “bulk flow” theory, is driven by the rhythmic pulsations of the brain parenchyma with each cardiac cycle.12,13,14
Much evidence currently exists that communicating hydrocephalus develops not due to impaired CSF absorption, but rather due to impaired compliance of the subarachnoid space in response to the rapid migration of CSF with each systolic event.10,14,15,16 Under physiologic circumstances, the brain expands during cardiac systole, causing local increases in ventricular CSF pressure that drive CSF into the spinal subarachnoid space, where the thecal sac expands to accommodate the increased CSF volume. The thecal sac thus normally serves as a buffer for the elevated CSF pressure occurring physiologically during systole. The compliance of the thecal sac is also thought to maintain patency of the venous side of the cerebral capillaries (through CSF “backpressure” exerted on the compressible outlets of the bridging cortical veins), allowing arterial blood within the cerebral arterial capillaries to travel unimpeded to the cortical veins and dural venous sinuses.14 Impaired ability of the thecal sac to tolerate increases in CSF volume during systole is thus theorized to result in poor outflow of blood from the cerebral capillaries. The result is an elevated intracranial pulse pressure amplitude (through breakdown of the Windkessel effect) and increased transmission of this elevated systolic pulse pressure to the brain tissue itself (due to self-compression of the parenchyma against the ventricular system), thereby resulting in a pulsatile, outwardly directed, transmantle pressure gradient which distorts the brain, eventually leading to the ventricular dilatation observed in communicating hydrocephalus.14,17 The distortion of brain parenchyma by repetitive pulsatile stress also compresses the cerebral vasculature, theoretically leading to ischemic changes and further reduction of intracranial compliance. Experimental animal models have indeed documented the development of communicating hydrocephalus after implantation of pulsatile balloons within the ventricular cavities,18 and intracranial-pressure monitoring studies have confirmed that patients with communicating hydrocephalus exhibit a substantially greater intraventricular pulse pressure,19 but the proposed transmantle pressure gradient has not been successfully captured or documented.20
The impaired compliance of the thecal sac buffer mechanism referred to in the above theory has been postulated to occur due to occult obstruction of CSF flow within the subarachnoid space (particularly at the cervicomedullary junction or within the basal cisterns) which may arise due to inflammatory changes (e.g., adhesions) seen after such insults as intracranial hemorrhage, infection, or leptomenigeal neoplastic proliferation, among others. Elevated intracranial pulse pressure is thought to play a similar role in iNPH, albeit due to primary changes of arterial hypertension, ectasia, atherosclerosis, and microangiopathic disease with which iNPH is often associated,14,16,21 or due to occult stenosis or obstruction of the aqueduct and/or basal cisterns,22 rather than reduced compliance of the thecal sac and venous capillary system.
10.3 Clinical Features
The neuropsychologic consequences of hydrocephalus are vast in scope and variety. Communicating hydrocephalus, like obstructive hydrocephalus, may lead to a multitude of clinical symptoms, due to cerebral ischemia (brought about by distortion and compression of the cerebral vasculature), demyelination, periventricular white matter edema, and damage to the cognitive, visual, motor, and awareness centers of the brain, among others. The clinical presentation in an individual case will vary with patient age as well as the degree of ventricular enlargement and of ICP elevation.
While obstructive hydrocephalus frequently presents in an acute manner with symptoms of elevated intracranial pressure (e.g., headache, nausea, vomiting, altered consciousness, and papilledema), communicating hydrocephalus often develops insidiously and may present with a wide variety of symptoms. Neonates and small children frequently present with rapidly enlarging head circumference, splayed cranial sutures, tense fontanelles, and failure to thrive.23 School-age children may present with vomiting, headache, and lethargy, or declining language function, motor and visuospatial skills, and attention and executive function.16 Adults with communicating hydrocephalus typically present with deficits in cognition, motor skills, and executive function that may be easily mistaken for sequelae of dementia. iNPH, for example, classically presents in the sixth to eighth decades of life with gait apraxia, progressive cognitive dysfunction, and urinary incontinence in the absence of elevated ICP (Hakim-Adams syndrome), but presenting symptoms are known to vary greatly, making the disease difficult at times to distinguish from other neurodegenerative conditions and subcortical dementias.5,24,25,26
10.4 Diagnosis and Neuroimaging
The diagnosis of communicating hydrocephalus is made primarily through a combination of clinical presentation, physical exam findings, and neuroimaging. Computed tomography (CT) and/or magnetic resonance imaging (MRI) of the brain classically reveal a “four-ventricle” pattern of ventriculomegaly (i.e., all four ventricles are enlarged, although lateral ventricle enlargement is often proportionally greater10 and fourth ventricular dilation is occasionally absent)16,27 without evidence of obstruction to intraventricular flow (e.g., aqueductal stenosis). T2-weighted MRI hyperintensities or CT hypodensities are commonly seen in the periventricular white matter adjacent to the lateral ventricular horns in long-standing communicating (or obstructive) hydrocephalus, often said to be due to “transependymal CSF flow” (although the origin of these findings is controversial). Some authors have reported that patients with communicating hydrocephalus will exhibit an exaggerated flow void anterior to the aqueduct of Sylvius on sagittal T2-weighted MRI (corresponding to higher CSF flow volumes and velocities through the aqueduct),28 dilation of the aqueduct itself (the pars posterior, in particular),29 or elevated CSF stroke volume within the aqueduct on phase-contrast MRI,30 among other findings. Others have reported a substantial weakening of the CSF stroke volume near the cervicomedullary junction on phase-contrast MRI performed in adults with communicating hydrocephalus,31 although this finding is not universally seen. Additional imaging findings may vary with differing etiologies of communicating hydrocephalus. Imaging studies in hydrocephalus after tuberculous meningitis, for example, may reveal enhancing exudates or adhesions in the basal cisterns and leptomeninges, parenchymal enhancement, or an associated tuberculoma.32
The role of neuroimaging has become especially prominent in cases of suspected iNPH, a diagnosis made particularly difficult by the variety of clinical presentations and courses, striking similarities to other disease processes prevalent in the same age group, and a lack of consensus regarding preferred terminology and diagnostic methods.22,26 The Evan′s index (the ratio of the distance between the most lateral aspects of the frontal horns and the distance between the inner table of the cranium on the same axial image, Fig. 10.1 ) is frequently utilized in the diagnosis of iNPH, with values of ≥ 0.3 being consistent with—but not sufficient for—the diagnosis of iNPH.22,26 The Evan′s ratio has not, however, proven useful in predicting successful outcomes with CSF diversion.22,26 The callosal angle (Fig. 10.1), a measure of the angle between two lines drawn parallel to the superomedial surfaces of the lateral ventricles on coronal MRI at the level of the posterior commissure, is another frequently employed radiographic tool in the diagnosis of iNPH, said to be < 90º in NPH and > 90º in ventriculomegaly related to Alzheimer′s disease or other dementias, with lower values showing the best response to ventriculoperitoneal (VP) shunting in some series.33 These radiographic findings are also often combined with evidence of ICP within the expected range for NPH (5–18 mm Hg, as assessed by lumbar puncture or other means26) and clinical improvement after a trial of CSF drainage to improve diagnostic certainty.

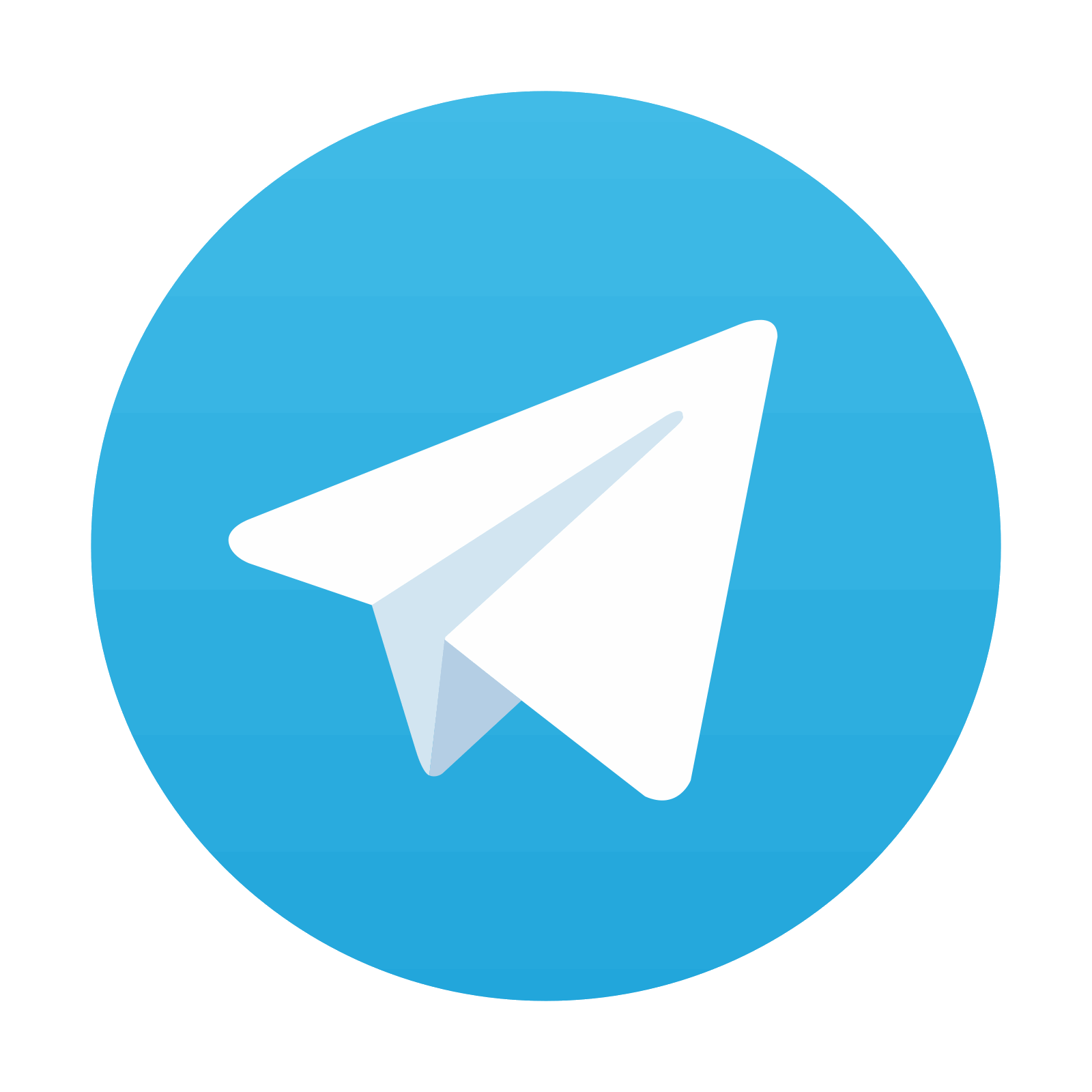
Stay updated, free articles. Join our Telegram channel
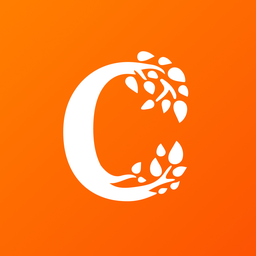
Full access? Get Clinical Tree
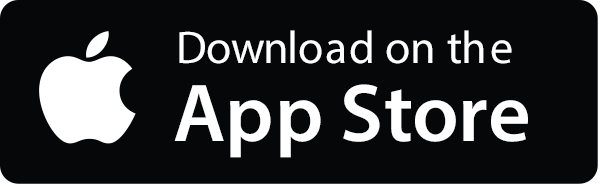
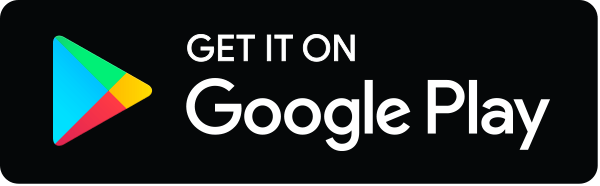