11 Cerebral Perfusion
Abstract
Lack of blood flow and substrate delivery to the brain results in brain ischemia and, if not compensated, infarction. The brain should maintain cerebral blood flow, which is dependent upon many issues of resistance and supply. The brain tissue resists the flow when there is edema, or receives decreased flow from poor blood viscosity or vessel constriction. The supply of blood flow to the brain is dependent upon the perfusion pressure reaching it. The cerebral perfusion pressure is dependent upon the function of the cardiac system. Too low blood pressure leads to ischemia; even one episode of hypotension in severe head injury can double the morbidity and mortality. Likewise, too high blood pressure will push through a damaged blood–brain barrier and cause further edema and damage.
Case Presentation
A 20-year-old man presents after a fall from a second-story window with obvious contusions to his chest, abdomen, and head. At the scene of the accident, he does not open his eyes or talk, and he exhibits extensor/decerebrate posturing. His pupils are equal and sluggishly reactive. There is no obvious distension or rigidity of the abdomen. His blood pressure is 90/50.
See end of chapter for Case Management.
11.1 Introduction
In light of any injury to the brain, whether it is due to a trauma, ischemic or hemorrhagic stroke, metabolic derangements, or neoplasm, it is imperative that hemodynamics be managed in a way that maintains adequate cerebral perfusion. Fortunately, the brain possesses some innate ability to respond to inadequate cerebral perfusion, though it is not a perfect system by far. To fully appreciate these mechanisms and gain insight into interventions that help us to maintain cerebral perfusion, it is first necessary to understand the underlying anatomy of the blood–brain barrier, circumventricular organs, and the mechanics of cerebral blood flow (CBF) and autoregulation.
11.2 Blood–Brain Barrier
The brain capillary varies from the systemic capillary by several unique features; these establish the blood–brain barrier. The brain capillary endothelial cells are the distinguishing elements of the blood–brain barrier. The brain capillary endothelial cells are not fenestrated, lack intracellular clefts, and are closed by intercellular tight gap junctions. The tight gap junctions of the brain capillary endothelial cells assist in keeping substances out of the brain interstitial space. The body systemic capillary endothelial cells are not in contact with one another; they do not make a circumferential ring barrier but instead have multiple fenestrations that allow fluid to pass freely (► Fig. 11.1). 1

The circumferential ring of brain capillary endothelial cells regulates which substances reach the brain’s interstitial space, the supporting astrocytes, and the neurons. In an intact blood–brain barrier, all substances pass through the capillary endothelial cell and the other regulator, the choroid plexus epithelial cell. The blood–brain barrier regulates the biochemical, immunological, and electrical passage of material into the brain. The tight gap junctions, the strong intercellular connections, have high electrical impedance, preventing ions from passing. The capillary endothelial cells exclude plasma proteins from the brain interstitial space because the endothelial cells have very little pinocytic vesicles to transport the proteins and contain unique cerebral enzymes, cellular channels, and transport systems (► Table 11.1). The brain capillary endothelial cells require large amounts of energy to serve as the complex regulatory interface between the brain and the blood circulation. The brain capillary endothelial cell energy-dependent transport organizations have three to five times the amount of mitochondria as the systemic capillary cells. Maintaining the blood–brain barrier’s unique functions, the brain capillary membrane has a different structural surface, and its lack of permeability is assisted by pericytes and astrocytic foot processes.
Tight gap junction | Different membrane structure |
Basement membrane | Neuronal enzymes |
Minimal pinocytic activity | Transport mechanisms |
High mitochondrial content | Pericytes (also seen in systemic circulation) |
High electrical impedance | Astrocytic foot processes |
The pericyte or perivascular cells are not unique to the brain; they are found partially wrapping systemic capillaries and venules in endothelial cells. The selective permissiveness into the brain interstitial space is not present in circumventricular organs, including the neural hypophysis, subfornical organ, median eminence, area postrema, and pineal gland (► Table 11.2). 1 , 2 , 3
11.2.1 Circumventricular Organs
The circumventricular organs represent structures in the brain where the blood-brain barrier allows for communication between the central nervous system and peripheral blood flow. These structures are highly vascular and can be divided into sensory and secretory organs (► Fig. 11.2, ► Table 11.2) 1 , 2 , 3

11.2.2 Brain Interstitial Circulation
In the normal state, for substances to pass from the blood to the brain interstitial space, they must pass through the capillary endothelial cell. The brain capillary endothelial cell allows certain compounds to pass with relative ease into the brain interstitial space via transcellular diffusion or ubiquitous transport mechanisms. These substances are lipid soluble, low molecular weight of ~ 500 daltons or less, nonpolarized, and not bound to proteins. Such substances include glucose, low-density lipoproteins, transferrin, bromide, morphine, and bile salts. These substances when not actively transported must still diffuse down a concentration gradient from blood to capillary endothelial cell to brain interstitial space. The blood–brain barrier is not permeable to ions and amino acids, and the exact type of permeability to water is controversial. Water does not enter through a lipid membrane but must pass through selective water channels regulated by aquaporins. The blood-brain barrier excludes water-soluble molecules with a molecular weight greater than 180 daltons. It is not permeable to vital dyes, epinephrine, curare, bile pigments, and fluorescein. Substances that enter the brain interstitial space fluid could alter nervous system function. These substances pass from the blood–brain capillary to the interstitial space, where they may be absorbed by neurons, glial cells, or others; they then pass through, but sometimes around, subependymal glial cells and ependymal gap junctions into the cerebrospinal fluid (CSF) of the ventricles, cisterns, and Virchow–Robin spaces, where they eventually circulate back into the blood via the arachnoid villi. 2 , 3 , 4
11.2.3 Blood–Cerebrospinal Fluid Barrier
The choroid plexus epithelial circumferential junction actively secretes substances into the CSF. The blood–CSF barrier is similar to the blood–brain barrier of the capillary endothelial cell. Substances dissolved in the choroid capillary blood pass more easily through the choroid endothelium, are selectively passed by the connective tissue of the choroid plexus, and then are regulated by the choroid epithelium. The choroid epithelial cells contain circumferential junctions, which must secrete CSF into the ventricle. The CSF acts as a sink for the brain interstitial fluid, with a gradient of substances carrying components away from the interstitial space.
11.2.4 Blood–Brain Barrier Disruption
Diseases of the nervous system disrupt the blood–brain barrier, resulting in most of the devastation. The barrier is disrupted by hypertension, hypercapnia, hypocapnia, trauma, hyperthermia, chemicals such as intraarterial lactated Ringer’s solution, mannitol, leukotriene C4, nitric oxide synthetase, arabinose, and lactamide, as well as in the center of tumors, but necessarily in the periphery. The disrupted blood–brain barrier leads to brain edema, accumulation of fluids and electrolytes, increased cerebral volume, increased intracranial pressure (ICP), cellular swelling, and cell death. There are many studies of brain edema in a variety of pathologies. However, there is a clinical situation that, when thoroughly understood, will clarify most questions about the blood–brain barrier and cerebral edema. That situation is head trauma.
11.2.5 Vasogenic Edema
At the moment of impact, the blood–brain barrier’s tight gap junctions are stretched, which essentially turns the brain capillary for ~ 30 to 60 minutes into a sieve, leading to vasogenic edema. There is accumulation of serum, albumin, and proteins. The opening is also prolonged for an unknown duration by hypoxia and for a single time of 6 hours by a hypertension surge. The mechanical disruption traumatizes microvessels, cells, and cellular organelles, releasing calcium, ornithine decarboxylase, and free radicals. Sustained increases of free radicals meant to oxidize toxins will disrupt the cellular membrane. An increase in intracellular and extracellular stores of calcium will be sustained by hypoxia.
The activity of ornithine decarboxylase is increased 2,000% due to upregulation of its messenger ribonucleic acid (mRNA). 5 It acts to decarboxylate ornithine forming putrescine, which is then converted into spermidine, then finally into spermine. All three are released immediately after cellular injury because of the necessity for regeneration, development, cell growth, and neuronal survival. Putrescine accumulation induces shrinkage in cerebral microvessels after trauma, opening the blood–brain barrier and increasing vasogenic edema. Putrescine can be increased by isoflurane and decreased by ketamine.
Bradykinins are released, which, with other pathway products, increase calcium at the time of mechanical disruption. This calcium increases calcium-calmodulin and calcium protein kinase C and opens the blood–brain barrier.
The disrupted blood–brain barrier is stabilized by dexamethasone, copper, zinc, superoxide dismutase, serotonin antibodies, and progesterone.
11.2.6 Cytotoxic Edema
The most common type of edema after head injury, cytotoxic edema, occurs in the cell 30 to 60 minutes after insult as a response to the intracellular accumulation of the toxic levels of lactate, hydrogen-ion acidosis, potassium, oxygen free radicals, and glutamate, among others. The sodium potassium adenosine triphosphatase (ATPase) pump fails secondary to depletion of cellular energy, allowing sodium to build up intracellularly and drawing in water. Hydrogen accumulation is the most common reason for buffering the cell by increasing the water content. The cell osmotically swells, reacting to the increasing glycogen granules that are sequestered to provide more substrate to the tricarboxylic acid (TCA) cycle. The additional energy is required to supply cellular active transport mechanisms, including the following:
Sodium potassium ATPase.
Sodium potassium chloride cotransporter.
Calcium-activated potassium channels.
Sodium hydrogen antiporter.
Chloride bicarbonate exchanger.
Carrier-mediated transport of nutrients.
Receptor-mediated transport of peptides.
As the cellular membrane starts to fail, glutamate is released, increasing cellular metabolism, which, under its current anaerobic conditions, leads to further lactate production and, without utilization, accumulation and then to failure of energy metabolism and cell death. 4
11.2.7 Interstitial Edema
Interstitial fluid drains down the gradient into the CSF sink. That sink starts to become clogged with toxins and proteins. CSF production is increased to buffer the brain interstitial fluid acidosis runoff by upregulation of the chloride bicarbonate exchanger. One method to decrease interstitial edema and cytotoxic edema (the major contributor to traumatic brain injury) is by decreasing the sink into which brain fluid drains. Decreasing the CSF in the ventricles does this; decreasing the pressure of the intracranial contents does not.
There is no silver bullet to treat brain injury. Fortunately, the brain can adapt and recover from multiple insults. Brain injury occurs because several innate brain systems fail to compensate for the overwhelming insult. Currently, there are experimental chemicals that are available to ameliorate different types of edema (► Table 11.3). 6
The aim of aggressive early treatment is to prevent ischemia. Glucose and glycogen are depleted in 4 minutes; there is increased metabolism in the layer surrounding the ischemic penumbra, the brain temperature increases, metabolism increases, and brain tissue PO2 drops to less than 20 mm Hg, favoring anaerobic respiration. Anaerobic respiration causes lactic acidosis and brings hydrogen into the cell. Lactate accumulation approximates glucose stores at the time of ischemia. The cell swells in response to increasing levels of hydrogen. Multiple neuromodulators stimulate cyclic adenosine monophosphate–dependention transport, bringing more hydrogen into the cell. The ischemia leads to the cellular pH dropping to 6.0 to 6.4, inhibiting mitochondrial respiration and the ability to sequester calcium. When the cell is deprived of energy and oxygen, calcium stores are released, stimulating phosphorylation of protein kinase C and further increasing intracellular hydrogen in a vicious cycle, leading to degradation of cell membranes. Therefore, increasing CBF in excess of edema is necessary to increase oxygen delivery.
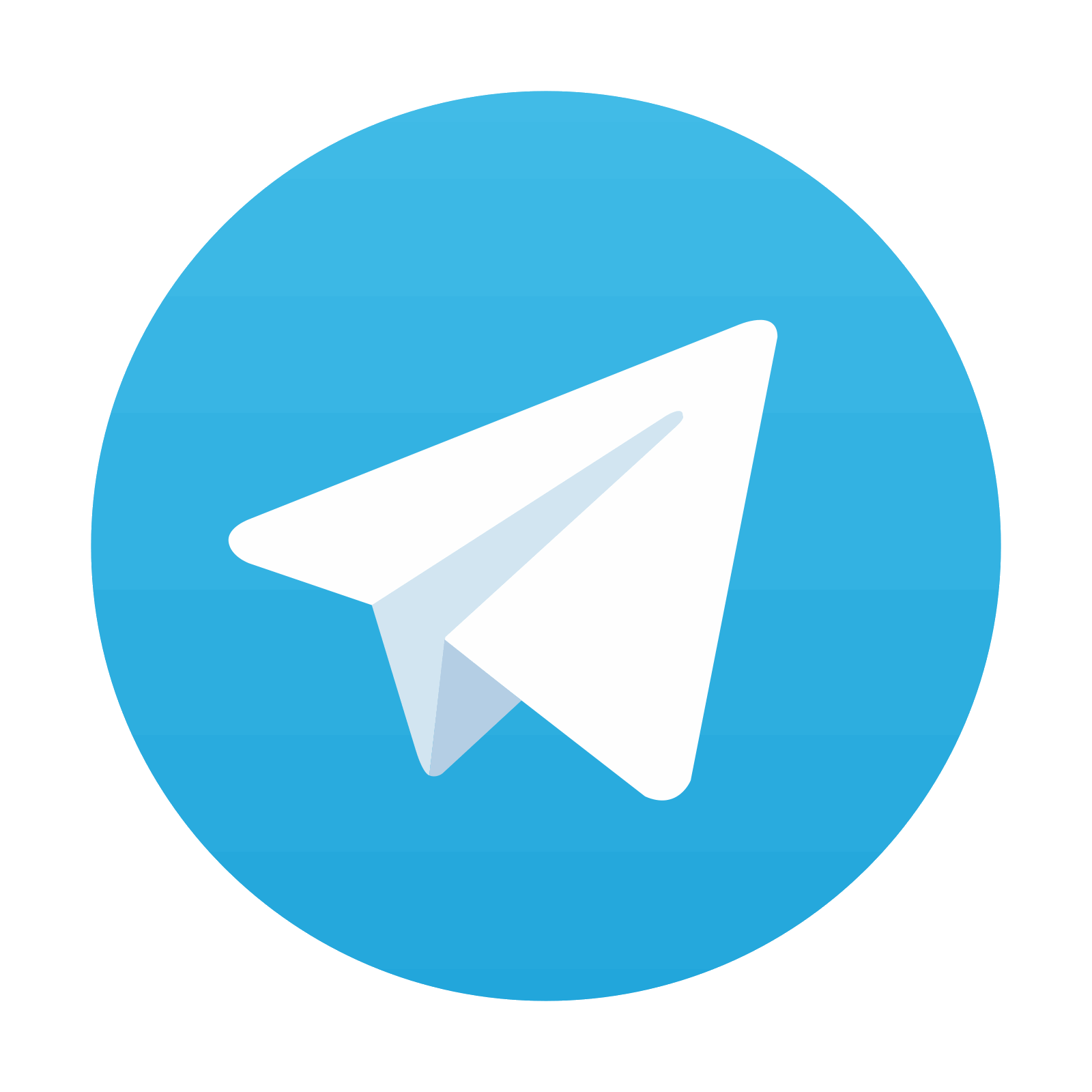
Stay updated, free articles. Join our Telegram channel
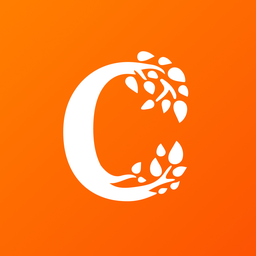
Full access? Get Clinical Tree
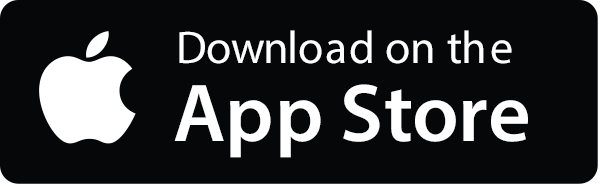
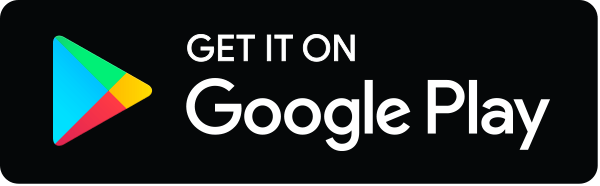
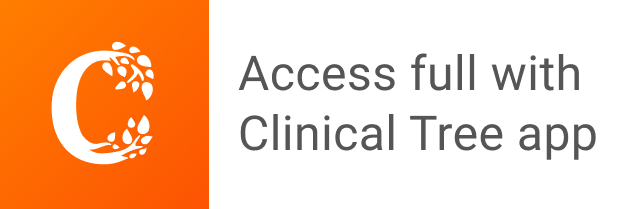