12 Imaging of the cerebrospinal fluid circulation
Adult Hydrocephalus, ed. Daniele Rigamonti. Published by Cambridge University Press. © Cambridge University Press 2014.
Imaging of flows
Magnetic resonance imaging (MRI) is the preferred medical technique for brain and central nervous system investigations. This technique is sensitive to the motion of hydrogen atoms that represent the main component of biological fluids. These displacements of protons are the source of prevalent artifacts in morphological MR imaging: depending on the motion velocity of the fluid elements present in the considered slice, phenomena known as inflow and outflow artifacts modulate the intensity of the impacted voxels. The example of the fast CSF flow induced artifact in the aqueduct of Sylvius (flow void), well known by radiologists as evidence of a permeable aqueduct on T2-weighted images, can also be used to qualitatively point out the hyperdynamic aspect of specific hydrocephalus pathologies.
The understanding and exploitation of this type of artifact allowed the development of time-of-flight MR angiography. These sequences are particularly sensitive to the signal enhancement induced by the uninterrupted inflow in the slice of “fresh” blood that did not undergo earlier magnetization flip and presents a full magnetization at the origin of the observed enhanced signal.
However, morphological imaging methods do not allow quantitative studies of blood flow.
The phase-contrast MRI (PC-MRI) technique, based on the classical gradient echo sequence allowing fast imaging, is very sensitive to the motion of biological structures. It can be useful to quantify liquid flow rates in order to detect slow or absent flows. This method detects the magnetization’s phase-shift of spins moving through magnetic field gradients (or alignment of individual spins relative to one another) with respect to static spins. Measurement of these phase-shift effects makes it possible to reconstruct images composed of flow sensitive pixels, where the pixel intensity reflects the local velocity [1].
The quality of PC-MRI acquisitions is dependent upon magnetic field inhomogeneities and local susceptibility artifacts. In order to cancel out the effects of external sources of spin dephasing, two gradients with the same magnitude but opposite direction are applied (bipolar gradient). Each of the gradients will shift the phase of spins within the tissues. In stationary tissues, the effects of the second pulse will exactly reverse those of the first. Conversely, fluid particles moving through the acquisition plane (vascular or CSF) will experience a net phase-shift proportional to its velocity.
Usually, the acquisition plane is selected perpendicular to the assumed flow direction axis. The reconstruction process then gathers the data in two-dimensional phase images, in which each pixel intensity is related to the local flow velocity. Depending on the manufacturers of MR scanners, this intensity can directly correspond to the velocity value or have to be calculated using specific vendors’ information. In addition to the velocity maps covering the acquisition slice, phase-contrast MRI generally simultaneously acquires sets of T2-weighted amplitude images depicting the anatomy.
This technique was initially developed to image the beating heart and quantify flow velocities through cardiac valves and shunts. As heart motion is periodic, cardiac triggering or gating must be linked to the bipolar gradient echo pulse sequence [2,3].
The cardiac pulse can be recorded by using either the electrocardiogram (ECG) or the plethysmograph signal. The ECG signal is the most widely used in cardiac gating techniques because systole identification is very accurate using this method. The detection of the R-wave on the ECG signal serves as reference for automatic acquisition. Nevertheless, this technique is tedious and may require repeated electrode repositioning. In addition, as the ECG signal is sensitive to the magnetic field gradients, ECG-gated acquisition may induce a significant increase in the sequence duration. In contrast, the plethysmography method using a peripheral transducer, less prone to magnetic field gradients influence, can be easily handled.
MRI cardiac gating can be retrospective or prospective:
Prospective gating technique sets the number of temporal phases splitting the cardiac cycle (CC) according to the lowest estimated CC length, which may induce misrepresentation of the diastolic phase.
Retrospective gating technique is preferred because the number of temporal frames is initially set, reducing signal discrepancies between the first and final phases of the CC. In clinical practice, 32 images per cycle is a good compromise between temporal resolution and acquisition time. The number of temporal frames covering the CC depends on echo time (TE), repetition time (TR), and flip angle. By using gradient refocused echo sequence, short TR, and low flip angles, the temporal resolution can be significantly increased and the duration of the sequence reduced [4].
Depending on the MR apparatus manufacturers, PC-MRI sequence can be associated with acceleration methods such as parallel imaging, partial field of view reconstruction, or view sharing techniques (e.g. number of views per segment). Nevertheless, these techniques reduce the accuracy and signal to noise ratio of images.
The main parameters that can potentially influence the quality and the accuracy of flow imaging are briefly recalled below.
Phase encoding is related to the velocity of spins within the limits of ±180°. If the absolute phase-shift is higher than 180°, phase wrapping will occur and the measured velocity will be underestimated. It is thus necessary to fit the velocity scale to the probable maximum flow velocity in the vessel. This scale factor is the velocity encoding parameter (Venc), which would correspond to a phase-shift of 180°. The Venc parameter sets the range of measurable velocities (–Venc, +Venc) without phase wrapping. The previous estimation of the extreme values of the detected velocities can therefore increase the measurement accuracy.
If Venc is lower than the actual flow velocity, aliasing artifacts due to phase wrapping will be encountered in the studied vessel; they visually correspond to areas where discontinuities in pixel intensities appear. The more Venc is small, the more aliasing areas will affect the velocity map. If Venc is higher than the expected maximum flow velocity, the signal to noise ratio decreases concomitantly with the measurement’s accuracy. Then, Venc must be adjusted depending on the studied flows and anatomical levels. For example, blood velocities are different in internal carotid and in small epidural veins; in the same way CSF oscillations in the narrow aqueduct of Sylvius can be hyperdynamic compared to CSF oscillations in the large pontine cistern.
A grayscale is used to represent PC-MRI measured velocities. Static tissues, corresponding to null flows, will be represented with gray pixels. Hyper- or hypo-intense pixels correspond to areas where circulation occurs at a velocity close to Venc absolute value.
The slice can be positioned so as to be parallel to the direction of flow; in this case, results are not quantitative but rather give a qualitative overview of the anatomical regions where fluid motion occurs (see Figure 12.7b, c). In order to record quantitative flow data, the acquisition slice must be perpendicular to the presumed direction of the flow (see (Figure 12.3).
If the plane is oblique with respect to the flow axis, each pixel velocity will be underestimated. Nevertheless, as the vessel section area is then proportionally overestimated, the flow rate (Flow = Velocity × Area) is theoretically preserved but spatial resolution limits generate a significant decrease in accuracy.
Main PC-MRI parameters usually encountered in cerebral acquisitions:
Signal to noise ratio can be improved by increasing the number of excitations (or averaging) at the expense of the duration of the acquisition. The spatial resolution and the subject’s cardiac frequency also influence the temporal length of the PC-MRI exam; this duration can vary from less than a minute to more than 10 minutes.
The best possible protocol results from a compromise between reasonable time acquisition for clinical exam and reasonable accuracy to suit specific “flow questions”: PC-MRI protocol could be different if the question is simply “Are there hyperdynamic CSF oscillations in this large aqueduct?” or precisely “What is the accurate value of CSF net flow in this very narrow aqueduct?”
Pelc et al. studied the cine PC-MRI sequence in terms of blood flow quantization within deep vessels (portal trunk) in dogs. The values measured using MRI were compared to an invasive Doppler echography technique (the Doppler probe was directly inserted in the studied vessel) [5]. This comparison exhibited a strong correlation between the two methods. The authors concluded that MRI would probably be able to quantify blood flow circulation in deep vessels in humans.
Buonocore and Bogren established the factors influencing the measurement accuracy of the PC-MRI sequence. According to their results, the slice thickness, the encoding velocity, and the temporal resolution as well as the choice of TR, TE, and flip angle, were parameters in which suitable settings were paramount in order to reach an acceptable measurement accuracy [6].
Greil et al. studied, in vitro, the different acquisition parameters and their potential effect on the velocity measurement accuracy. They concluded that the key factor was the spatial resolution of the image modulated by the field of view and the matrix [7].
During an in vitro study using an MRI phantom, McCauley et al. validated the PC-MRI sequence for velocity measurements in unsteady flows. After comparison of MRI to standard Doppler velocimetry, they showed that the second technique was significantly less accurate [8].
Enzmann et al. and Wahlin et al. showed the good reproducibility of the PC-MRI technique for quantization of weak liquid oscillations such as those encountered at the aqueduct level [9,10].
For all PC-MRI flow measurements, one must take into account the effects of eddy currents in all vendors’ scanners as they introduce a significant velocity phase offset. Sequence and protocol optimization is required to reduce this intrinsic source of errors [6,9,11,12].
As PC-MRI is a functional imaging method, the post-processing step is crucial. PC-MRI was at first mainly used for vascular flow quantization and many software packages were developed by MR vendors to measure blood flows in vessels. As blood flows are mainly unidirectional and vessels’ boundaries present simple geometrical shapes, several automatic algorithms were developed, allowing quick, reproducible, and accurate blood vessel segmentation [13]. CSF flow oscillations arise in complex anatomical regions such as subarachnoid spaces. Thus CSF flow studies require a specific CSF segmentation algorithm, which may explain why CSF flow investigations have not been widespread in clinical practice.
Balédent et al. and Alperin and Lee developed home-made flow software based on the analysis of CSF and blood velocity changes during CC. Using this tool, dedicated to both blood vessels and subarachnoid space (SAS) segmentation, the authors showed that one could extract velocimetric data of blood and CSF flows in a reproducible way (inter- and intra-observer), allowing an accurate, reproducible, and automatic segmentation of blood vessel and SAS lumens [14,15].
In this chapter, CSF and blood flow values were calculated using the PC-MRI post-processing software developed by Balédent et al. (software can be freely downloaded at: www.tidam.fr [14]). The methodology of this pixel-based software is focused on several robust frameworks that provide reliable results. The main ideas underlying the algorithm are described below.
In order to correct for possible phase offset, the average apparent velocity of a neighboring region of interest was first subtracted from the phase velocity [11,16]. The segmentation algorithm consisted of two successive steps: the creation of a new parametric image, obtained for all time frames, followed by the application of a threshold. In this new image, each pixel intensity depended on the magnitude of the fundamental frequency, i.e. the subject’s cardiac frequency. The magnitude of this spectral component was assessed using a fast Fourier transformation (FFT) for each pixel signal during CC. Regions exhibiting cardiac periodicity, such as CSF areas, were highlighted on the new image where pixel intensities were characterized by their dependence of flow on heart rate. A threshold was applied to the parametric image so as to extract all the pixels with a velocity signal at the cardiac frequency above a visually selected threshold. This threshold usually made it possible to differentiate tissue with low fundamental component magnitude and identify CSF pulsating motion, which has a large fundamental component magnitude (see Figure 12.2). If CSF and non-CSF regions were adjoining, a phase threshold was then applied to identify actual CSF pixels.
Temporal normalization
All the temporal data were expressed as percentage of CC and the time of internal carotid systolic peak flow of an individual was used as time reference. This time shift was applied to all the velocity curves for each subject.
Aliasing correction
Aliasing pixels are characterized by their high velocity in the opposite direction to the average velocity of the segmented area. Corrected velocity values for such pixels were recalculated as Vcorrected = (2 * Venc – |Verror|) * (– |Verror|/Verror).
CSF flow parameters
The flow rate through a region of interest (ROI) is estimated as the product of the averaged velocity of the pixels within the ROI and the area of the given ROI. Stroke volume (SV expressed in µl/CC) corresponds to the mean of the two oppositely directed displaced volumes during the CC. This is the most prevalent parameter, often applied in the aqueduct of Sylvius to characterize CSF flow [16,17].
In Figure 12.1, a series of 32 phase images resulting from PC-MRI is depicted. Images were zoomed to clearly visualize CSF intensity changes during a whole cardiac cycle. The slice being studied was positioned perpendicularly to the spine at the C2–C3 level. In the first images, white pixels represent the flush of CSF in the subarachnoid spaces around the spine and black arrows show the main CSF compartments separated by spinal nerves.
Figure 12.1 Cervical CSF flow intensity changes during the cardiac cycle.
During CC, CSF pixel intensities gradually decrease toward gray color where the liquid velocities reach a null value. Then, the color progressively darkens toward black, which corresponds to the moment when CSF fills the cranium. Periods of CSF flushing/filling alternate, paced by the heart beat.
In these images, white arrows point at two regions showing the blood flow in the epidural veins, which are close the subarachnoid spaces. In this example, as epidural blood flow exhibits velocities up to 5 cm/s, the aliasing phenomenon (dark areas) arises in the vessel.
Available processing software, using automatic segmentation, makes it possible to quickly obtain a reproducible time evolution curve during the CC. After background correction using a manually drawn area, flow parameters such as CSF stroke volume are directly calculated (Figure 12.2).
Figure 12.2 PC-MRI post-processing. PC-MRI image of CSF flow at cervical level (C2–C3). The software extracts the outline of regions of interest (ROIs) and calculates flow curve. To correct for the phase offset due to eddy currents, the average apparent velocity of a static neighboring region throughout the cardiac cycle was subtracted from the phase velocity of the ROIs. This background region is manually drawn (white line) in a static anatomical region. Vascular vessels and CSF flows from other anatomical levels are measured in the same way.
CSF and blood flow interaction
As CSF is an incompressible fluid, Pascal’s law indicates that, from a static point of view, any pressure variation in an elementary volume of the CSF compartment would thus be integrally transmitted at any point in the whole CSF volume.
The compliance of a compartment can be defined as the ratio of volume change to the corresponding pressure change. This parameter is an indicator of the distensibility of the compartment and corresponds to the ability to bear a pressure increase, resulting from volume fluctuation, without disruption of the structure function. When compliance is known, it should be possible to assess the magnitude of the CSF pressure required to bring the CSF compartment back to its initial volume after a volume change in one of the other intracranial compartments (blood or brain).
The cranio-rachidian skeleton and the meningeal dura mater can be considered to constitute a rigid undeformable structure in adulthood. In children, fibrous and elastic cranial sutures and fontanelles provide the cranial box with the flexibility necessary for nervous growth and development; these fontanelles usually close progressively from birth until the end of the second year in infancy. CSF and blood flow also change during this growing period [18].
In this rigid closed “box,” three compartments interact: the nearly incompressible vascular (arterial, capillary, and venous) and CSF compartments and brain parenchyma. Blood and CSF compartments are incompressible. Although the glial cells constitute a soft flexible embedding, the cerebral parenchyma is also usually supposed to be incompressible and needs the extracranial compliance distributed in the spinal canal and the dural sac.
The interactions between these various compartments consist of periodical liquid volume displacements and exchanges. Actually, the cranial box deals continuously with two imperatives. The first obvious requirement is an intracranial inflow of arterial blood at each cardiac cycle (CC), which is necessary to supply the brain with essential nutrients for regular cerebral functioning. The second requirement is to regulate the outgoing venous and CSF flows in order to maintain a stable pressure in the cranial box, as a significant increase in intracranial pressure might be harmful for the brain, hindering normal cerebral functioning.
In this context, PC-MRI is a valuable tool to investigate and quantify these CSF and blood flow interactions throughout the cardiac cycle. In the following parts of this chapter, normal adult hydro- and hemodynamics will be described. Then these results will be used as references to better detect cerebral flow alterations of hydrocephalus patients.
In healthy adults, MRI flow studies showed that the CSF flush venting from the intracranial SAS into the cervical spinal SAS, which had a high magnitude and elapsed very quickly, was the main lever control in instantaneous regulation of ICP. In contrast, the ventricular CSF flow represented less than 6% of the intracranial blood variation, and occurred at almost 20–25% of the CC duration. Therefore, it appeared that, in healthy subjects, the ventricular system plays a minor role in the dampening of the general systolic expansion [14], while the “CSF mobile compliance” [19] of the intracranial cavity depends mainly on the SAS. Additionally, the temporal analysis of flow curves tended to confirm previous theories about the relation between brain expansion and the ventricular pulsations. We [14] hypothesized that the early CSF flush through the fourth ventricle reflects the early infratentorial expansion, while the delayed CSF flush through the aqueduct, the third ventricle, and the foramina of Monro rather reflects the delayed supratentorial expansion. Finally, a close correlation between CSF aqueductal stroke volume and time point of jugular peak flow was found, which indicated a direct quantitative relation between brain expansion and ventricular pulsation. Based on these findings, we suggested a global modeling of both CSF and blood flow relationships throughout the different phases of the cardiac cycle. The description and understanding of these successive phenomena in healthy subjects would enable detailed and extensive volumetric analysis in clinical practice, in order to access pathological changes and to detect the perturbations of CSF dynamic patterns in several neurological disorders.
PC-MRI is a powerful tool to investigate CSF and blood circulation but its use in clinical practice requires careful selection of the anatomical part for investigation. Figure 12.3 displays an example of the four main regions explored in an efficient CSF flow protocol. In less than 10 minutes a global overview of the hydro- and hemodynamics of the craniospinal system is made possible.
Figure 12.3 Data acquisition. Sagittal scout view sequences were used as localizer to select the anatomical levels for flow quantification (a). The acquisition planes were selected perpendicular to the presumed direction of the flows. Sections through the aqueduct of Sylvius (b), pontine cistern and foramen of Magendie (c), and C2–C3 subarachnoid spaces level (d), were used for CSF flow measurement. By varying the velocity encoding (Venc), the same cervical section level (e) was used to measure vascular flows in left and right internal carotids (ICA), vertebral arteries (VA), and internal jugular veins (IJV). Black pixels represent the flows entering the slice, white pixels represent flows directed out of the slice, and gray pixels correspond to immobile tissues.
By applying this protocol in healthy adults, we observed that CSF and vascular curves presented comparable and reproducible oscillations even if amplitude variations in vascular and CSF flow due to age, anatomy, or physiology were present [20]. These variations are moderate compared with important alterations in CSF and blood flows induced by cerebral pathologies such as hydrocephalus, syringomyelia, and cerebral hemorrhage [17,21,22].
Figure 12.4 displays an example of normal CSF and blood flow curves during the CC in a 30-year-old woman. These curves are representative of flows measured in the healthy young adult population described in Table 12.1.
Table 12.1 Blood and CSF flow in healthy subjects, hydrocephalus stenosis patients before and after endoscopic third ventriculostomy (ETV), and normal pressure hydrocephalus (NPH) patients
N, number of subjects; CBF, mean cerebral blood flow; cervical CSF, CSF stroke volume at C2–C3; ventricular CSF, CSF stroke volume in the aqueduct or in the aperture of the third ventricle for patients who underwent ETV.
Figure 12.4 CSF and cerebral blood flow during the cardiac cycle in healthy adults (see text for full explanation).
The knowledge of individual arterial flow curves makes it possible to calculate the cerebral blood inflow, Figure 12.4a, which is the sum of left and right internal carotid flows with the vertebral artery flow. Mean cerebral arterial blood flow was close to 700 ml/min in adults.
The cerebral venous outflow resulted from the sum of left and right internal jugular flows and epidural veins flow. In order to take into account unmeasured small veins, the amplitude of the measured cerebral venous waveform was corrected, so that the average of the corrected cerebral venous flow rate was equal to the previously measured average arterial inflow (Figure 12.4b). Cerebral venous flow is not steady during the cardiac cycle but presents noteworthy oscillations resulting from arterial pulsations, intracranial pressure, and probably also from heart suction. It is interesting to note that arterial and venous flows exhibit an equivalent amplitude during the diastole period (x-axis: 9–12) and just prior to the systolic peak flow (x-axis: 24–26). The equilibrium period (called “brain equilibrium pressure”) when arterial inflow and venous outflow are almost balanced corresponds to the moment when CSF reverses its flow at the cervical level, as displayed in Figure 12.4c.
Indeed, the curve depicted within this figure represents the intracranial arterio-venous flow difference (subtraction of venous flow from arterial flow) throughout the CC: a positive systolic peak flow (arterial flow > venous flow) and two diastolic negative bumps (venous flow > arterial flow) are shown. The positive values of this calculated curve represent an increase in blood volume whereas negative values are related to a decrease in blood volume. The integration of the positive part of the curve represents the intracranial blood volume change during the CC. The arterio-venous curve, reflecting the pulsatility of the brain during the cardiac cycle, is the main source of intracranial pressure (ICP) fluctuation during the CC. Actually, ICP amplitude is also dependent on CSF volume changes in the cranium as well as intracranial compliance.
As can be observed in Figure 12.4c, arterio-venous difference and CSF flow measured at the cervical level are oscillating in opposite phase with similar amplitudes: positive flow values correspond to CSF flushing through the spinal canal and negative values correspond to CSF filling the cranium. CSF seems to balance the vascular expansion so as to minimize the potential increase in ICP during the systolic phase because arterial inflow is not instantaneously drained by the venous system.
In the same way, summation of CSF and vascular flow curves are calculated to obtain the intracranial volume change (IVC) during a CC [23]. As a function of the craniospinal compliance, IVC generates pressure changes in the equilibrium ICP. The pressure fluctuations due to volume variations were determined by Marmarou’s monoexponential function [24]:

where P is the instantaneous intraventricular pressure, P0 is the resting equilibrium pressure, and k is the elastance (inverse of compliance) factor. This highlights the major role of CSF in the control of ICP during the CC.
The intracranial compartment can be assimilated to a “black box” that converts the arterial input signal into venous and CSF output signals. The ICP temporal evolution controls the cerebral blood perfusion. Then, monitoring of ICP during the CC is a key parameter for understanding and diagnosis of diseases related to CSF flow alterations.
The PC-MRI CSF–blood approach may help to estimate the ICP waveform but a missing link still remains. Although Alperin [25] proposed, through various assumptions, a PC-MRI method to assess intracranial elastance and ICP in six baboons, no paper in the literature has demonstrated or corroborated this finding in large human populations or in specific phantom models. In the future, this scientific void may be filled using specific phantom models of the craniospinal system [26].
Figure 12.4d displays the CSF oscillation in the aqueduct during CC; the green area represents the aqueductal CSF stroke volume. In comparison with the cervical level, the amplitude of aqueductal CSF oscillation is nearly 10 times smaller and presents a significant phase-shift [14]. The volume of CSF in intracranial SAS is around the value of CSF ventricular volume. Nevertheless, as the long and narrow tubular aqueduct of Sylvius imposes a substantial resistance to flow, the impact of ventricular CSF flow in ICP amplitude change during CC is then very low in healthy subjects. It highlights the major influence of CSF at the intracranial SAS level (pontine, cerebellar cisterns), which oscillates between the cranium and the spinal canal.
From the CSF and blood flow curves measured in a healthy population, a chronological sequence of events has been generated. Figure 12.5 shows how the fast systolic blood inflow impacts on the volume exchanges between the compartments. A more global and synthetic depiction is displayed in Figure 12.6.
Figure 12.5 Chronological sequence of cerebral blood and CSF peak flows during early phase of the cardiac cycle. The cardiac systole can be divided into five phases according to the CSF or blood peak flows; the time of appearance of these peaks and their magnitude (in ml/s) are depicted. During the first phase, the cerebral blood volume instantaneously increases because of the vertebral and internal carotid systolic peak flows. The quickest way to absorb this and to regulate brain volume expansion is to flush extracerebral CSF into the spinal SAS (second phase), thanks to the spinal and dural sac compliance. During the third phase, a significant jugular output flow occurs and CSF progressively flushes out of V4; later (fourth phase), aqueduct, V3, and Monro flushes appear, with a very small contribution to the volume regulation. During the fifth phase, after a transient equilibrium state, brain CSF filling occurs and the reverse sequence begins.
Figure 12.6 Diagram of CSF and blood flow. The large and rapid amplitude change in cerebral arterial input flow [1] increases the brain volume over the intracranial subarachnoid spaces (large red arrows). In these areas, resistance to flow is low and as CSF viscosity is low, CSF is quickly displaced out of the cranium toward the compliant spinal canal [2]; ICP increase is therefore limited. Nevertheless, this first CSF response is scanty and has to be supplemented with the cerebral blood venous outflow [3]. Due to blood viscosity, this venous contribution is slower but brings a greater displaced volume. Finally, the pressure decreases at the brain periphery induce a CSF ventricular flow, out of the fourth ventricle and through the aqueduct of Sylvius, resulting in a small inner displacement of the brain directed toward the ventricles (small red arrows). After this series of flow events during the systolic phase of CC, arterial inflow equals venous outflow and cervical CSF flush stops. After this brief equilibrium pressure moment, venous heart aspiration increases the cerebral venous outflow, decreases ICP, and reverses the cervical CSF flow to fill the cranium and prepare the next cycle.
As CSF is mainly produced in the ventricular choroid plexus, we can rationally hypothesize that a residual net flow directed toward the fourth ventricle arises. Theoretically, the PC-MRI method could measure or approach this secretion [27] but many constraints such as spatial and temporal resolution, eddy currents [12], intermittent CSF secretion, or variable heart rate limit the chance to meet this challenge. New MRI sequences based on spin labeling technique seem more relevant to observe and measure the CSF net flow in the aqueduct [28]. The choroid plexus may also play a role in CSF absorption and is not the sole source of CSF production [27,29,30].
Imaging of CSF disorders
In clinical practice, the most commonly encountered CSF disorders observed using radiological images are hydrocephalus in the brain and syringomyelia in the spine. These two pathologies have a characteristic in common: CSF volume increases in the center of the nervous system, generating compression of the surrounding tissues.
The aim of the following section is to show how PC-MRI of CSF can help clinicians to diagnose and understand these disorders as well as how it can help neurosurgeons to guide treatment.
Hydrocephalus can be seen as an alteration of the CSF circulation between its production and absorption sites but also, in light of the previous section, as an alteration of its displacements between the various CSF compartments of the craniospinal system. Aqueductal stenosis, one of the common origins of hydrocephalus, can be induced by tumor compression detected using standard morphological MR imaging. Absence of flow void in T2-weighted images can be a good indicator of stenosis and 3D-SSFP (three-dimensional balanced steady-state free precession) sequence is available to investigate a wide range of CSF pathologies when traditional MRI sequences do not provide the desired anatomic information (Siemens TrueFISP, General Electrics FIESTA, and Philips balanced TFE) [31].
During our clinical experience, according to the morphological images, we have observed patients with suspected aqueductal stenosis showing CSF oscillations in the aqueduct and patients without evidence of stenosis demonstrating null flow in the aqueduct on PC-MRI images [32].
As a consequence of this observation, the PC-MRI protocol is performed in all cases of suspected aqueductal stenosis in our hospital. Low Venc (5 or 2 cm/s) is required to confirm the absence of CSF flow in the aqueduct (Figure 12.7).
Figure 12.7 Incomplete stenosis of the aqueduct of Sylvius. CSF MRI investigations in a 42-year-old woman presenting with hydrocephalus and syringomyelia. Control 3 months after foramen magnum surgery. (a) 3D-FIESTA image highlights CSF splitting and hyposignal in the aqueduct probably due to aqueductal stenosis. At the bottom of the image, hypersignal inside the spine indicates the beginning of a syringomyelia cavity. (b, c) Sagittal PC-MRI view with velocity sensitivity selected in the up–down direction. Image (b) corresponds to systolic phase with CSF flushing towards the spinal canal, CSF appears in white around the spine and in the subarachnoid spaces but not clearly in the aqueduct and the foramen of Magendie. Aliasing inside the pontine cistern corresponds to the blood flow in the basilar artery whose velocities are much greater than 5 cm/s. Image (c) corresponds to the diastolic phase with CSF filling the cranium. These sagittal images (b, c) cannot be used for quantitative investigation of CSF flow but allow a global qualitative view of the CSF circulation during the cardiac cycle. (d) Quantitative PC-MRI exam has been carried out at the aqueduct of Sylvius level with very low velocity encoding (Venc) to depict very small but real oscillations. When Venc was adjusted to 10 cm/s, no flow was detected. (e) PC-MRI shows also the functional pontine cistern, foramen of Magendie, and cervical subarachnoid spaces in the cervical canal (f). The stroke volumes of CSF during the cardiac cycle were calculated after post-processing and are written on image (a) at their respective levels. Results show that, though surgery established a normal CSF circulation between the cranium and the spinal canal, communication between the ventricles and the subarachnoid spaces remained abnormal.
Table 12.1 shows that hydrocephalus patients with aqueductal stenosis have an increase in CSF oscillations in the spinal canal probably due to the associated ICP increase.
Endoscopic third ventriculostomy (ETV) is an interesting surgical treatment for aqueductal stenosis pathologies. Nevertheless, the aperture made in the third ventricle (V3) is not characterized by the same hydrodynamic characteristics as the functional aqueduct. Indeed, CSF flow rate can be calculated by the ratio of pressure difference between the third ventricle and subarachnoid spaces to the resistance of the relevant channel (aqueduct or V3 aperture). These resistances, related to the radius and length of the fluid passage, are obviously not of the same order of magnitude in the geometrically different aqueduct and V3 aperture. It is then interesting to quantify CSF oscillations through the V3 aperture and to evaluate the impact of ETV on hydro- and hemodynamics.
After ETV, cervical CSF oscillations significantly decreased in aqueduct stenosis patients (Table 12.1). This decrease is probably associated with the decrease in ICP [33].
As was done in the aqueduct, the functionality of the third ventricle aperture has also been quantified using PC-MRI by computation of temporal flow curves in order to extract the associated stroke volume (Figure 12.8). Through this aperture, CSF presented oscillations with a temporal evolution similar to the one observed in volunteers’ aqueducts but amplitudes were greatly increased. No significant correlation was found between the V3 CSF oscillation and the aperture area, the cervical CSF oscillation, or the arterial flow amplitude.
Figure 12.8 CSF flow in the V3 aperture after ETV. (a) Sagittal T2-weighted image showing flow void (black arrow) in the aqueduct characterizing flow blockage. (b) CSF flow through the aperture performed by the neurosurgeon re-established CSF communication between the third ventricle and the subarachnoid spaces. (c) CSF oscillation through the aperture compared to aqueduct CSF oscillation calculated in a healthy population.
As noted for the aqueduct analysis, it is important to carefully position the PC-MRI slice; at the V3 level, it must be close to the roof and perpendicular to presumed direction of CSF flow. In the case of huge flow through the aperture, the presence of flow void in T2-weighted images can help in choosing the best slice location.
Another potential issue is the proximity of the basilar artery as blood can generate aliasing artifacts which can be difficult to differentiate from CSF flow.
The Venc factor used for the V3 acquisition varies from 5 to 15 cm/s, depending on the size of the aperture and the pressure distribution.
Quantization of CSF oscillations through the V3 aperture is very useful to control its permeability and to check the efficiency of the treatment. This could be useful also in understanding the physiological aspects of the surgery and may, in the future, help to select patients who finally need a shunt because other CSF flow alterations still persist.
Since its first description in 1965 [34,35], the syndrome of normal pressure hydrocephalus (NPH) has remained a diagnostic and therapeutic challenge. Paradoxically, despite the name of this syndrome, the ICP pulse waveform of chronic adult hydrocephalus patients is not so normal [36] and NPH can be a confusing name [37].
In 2005, an independent study group was assembled and addressed evidence-based guidelines for the management of NPH patients [38]. A part of this assessment examined the value of supplemental tests in the diagnosis of NPH and prediction of which patients would benefit from placement of a shunt. Among all of these tools (cisternography, ICP monitoring, tap test, external lumbar drainage, CSF resistive outflow Rout), PC-MRI seemed to be of potential interest through the evaluation of aqueductal stroke volume, but neither CSF flow void sign nor quantitative CSF flow velocity seemed to have significant diagnostic value. Greitz et al.[39] observed that aqueductal flows in NPH patients were 10 times greater than those in normal subjects. Luetmer et al. found the average flow rate in patients with NPH to be significantly higher than the flow rate in normal age-matched controls or in patients with Alzheimer’s disease [40].
Actually, the value of ventricular CSF was first suggested by the exaggerated aqueductal flow void on axial MRI scans. Jack et al. [41] noted that the increase in CSF flow dynamics at the level of the aqueduct and the fourth ventricle was correlated with NPH patients’ response to shunting. In contrast, although Krauss et al. [42] found a significant increase in the extension of the flow void in the aqueduct and the third and fourth ventricles in the NPH group, they did not find any correlation with the postoperative outcome.
The usefulness of stroke volume in the evaluation of postoperative prognosis was first suggested by Bradley et al. [17], but this was not replicated in the study of Dixon et al. [43] which found no significant correlation between increased aqueductal CSF flow and outcome following shunt. However, Dixon et al. as well as El Sankari et al. observed an increase in mean aqueductal CSF flow in patients who improved after shunting although they had a negative tap test [40,44].
Wagshul et al. [45] suggested a stroke volume ratio as a measure of hyperdynamic flows in NPH, reflecting a readjusted distribution of flows between the subarachnoid and the ventricular spaces as previously proposed by Balédent et al. [46]. They observed that cervical CSF flow was similar in NPH patients and healthy volunteers whereas ventricular flow was greater in the control group. Ventricular CSF represented 11% of the cervical CSF stroke volume in healthy volunteers, compared to 42% in NPH patients. Therefore, intracranial subarachnoid flow was dramatically reduced in NPH subjects. These results are in accordance with the findings of Kitagaki et al. [47], who observed that CSF volume in patients with idiopathic normal pressure hydrocephalus was significantly decreased in the subarachnoid spaces.
In healthy subjects, intracranial CSF mobile compliance depends predominantly on intracranial subarachnoid CSF pulsations. Conversely, in NPH patients, the ventricular pulsations play a major role in cerebral pressure damping during vascular brain expansion; ventricular dilation seems to be an adaptive response to the alterations of subarachnoid intracranial CSF pulsations (Figure 12.9). An increase in CSF flow is linked to a pressure difference increase as Czosnyka et al. observed an ICP amplitude increase during invasive pressure monitoring in NPH patients [36].
Figure 12.9 Schematic representation of CSF circulation in a healthy subject and chronic adult hydrocephalus. Red arrow represents arterial flow. Blue arrow represents venous outflow. White arrows represent CSF oscillation during cardiac cycle. In this model restricted CSF oscillations in the intracranial subarachnoid compartment increase CSF oscillations in the ventricle to maintain cervical CSF oscillations, then balance vascular expansion and preserve a large increase in ICP during the cardiac cycle.
In NPH, ventricular dilation may be related to ventricular wall modifications induced by an increase in the pressure difference between ventricular and extraventricular CSF, whereas in atrophic brain, ventricles are dilated because tissue is missing. When reliable diagnosis is difficult, CSF oscillation studies could be helpful to differentiate one state from the other.
As an example, Figure 12.10 demonstrates how PC-MRI can be used in patients presenting with ventricular dilation and clinical signs of NPH. PC-MRI results were compared to the reference values summarized in Table 12.1. Patient 1 (a, b) presented with normal CSF circulation indicating that the pressure differences which generate CSF flows were not altered: the observed ventricular dilation was probably due to atrophy rather than NPH. Patient 2 (c, d) presented with hyper-oscillations between all of the CSF compartments. CSF oscillations in the spinal canal were twice the normal value, indicating a probable increase in ICP amplitude and normal functionality of spinal spaces. Aqueductal CSF oscillations were six times the normal value and represented 30% of cervical CSF oscillations versus only 10% in the healthy subject. In this case, ventricular dilation was probably due to an increase in the amplitude of pressure difference between the CSF compartments during CC.
Figure 12.10 CSF flow in two patients with suspected NPH. (a, b) Morphological MRI of a 72-year-old woman with slowly progressive dementia, Parkinson syndrome, and a significant gait disturbance. (a) Her ventricles were dilated with hypersignal signs of transependymal resorption of CSF with significant atrophy. (b) The CSF stroke volumes from PC-MRI acquisition at the level of the aqueduct, the pontine cistern, the foramen of Magendie, and in the spinal canal at the C2–C3 level are displayed. Cerebral arterial inflow was 492 ml/min. A tap test was done and resulted in a negative response and post lumbar puncture headache. No surgical treatment was undertaken. (c, d) Morphological MRI of a 56-year-old woman with isolated gait disturbance. (c) T2 axial FLAIR image showing dilation of the ventricles without important signs of transependymal resorption. (d) CSF stroke volume is presented in sagittal T2-weighted MRI: flow void is important inside the aqueduct in accordance with the very large calculated stroke volume. Cerebral arterial inflow was 566 ml/min. Tap test response was positive, then the patient was shunted and a few days after surgery her walking had improved. CSF dynamics of the first patient (a, b) was in the range of normal values (Table 12.1) whereas the second (c, d) had greatly increased oscillations.
From a previous study on achondroplasia [48] and the observation of patients undergoing hydrocephalus associated with craniostenosis, Sainte-Rose et al. [49] concluded that an increase in the superior sagittal sinus venous pressure could be the cause of enlarged ventricles.
PC-MRI is also a good tool to investigate cerebral venous flows [50,51]. Bateman [52] explored the pulsatility of venous flows, showing that NPH patients exhibited a significant increase in pulsatility inside the straight sinus. Such a patient with hydrocephalus associated with jugular stenosis invites the question: “which is the chicken and which is the egg?” (Figure 12.11).
Figure 12.11 CSF and cerebral venous blood flow alterations in a hydrocephalus patient. MRI of a 32-year-old hydrocephalus patient suffering from severe headache and presenting with ventricular dilation (a, d). CSF stroke volumes in the aqueduct, foramen of Magendie, pontine cistern, and spinal spaces are displayed. (b,e) Venous angiography showed that cerebral venous outflow was restricted in jugular veins and predominantly in the posterior part (white ellipse). Axial vascular (c) and CSF (f) flow investigation confirmed the results. Cerebral arterial flow was 953 ml/s. The PC-MRI report pointed to a possible venous origin of hydrocephalus in this patient.
Coupling of the cardiovascular and cerebrospinal fluid (CSF) system is considered to be important in understanding the pathophysiology of cerebrovascular and craniospinal disease. A coupled cardiovascular and CSF system model was designed to examine the relation of spinal cord blood flow and CSF pulsations along the spinal subarachnoid space [53].
As hydrocephalus mainly results from a brain compression initiated by CSF flows, it seems logical to associate this flow-induced stress mechanism with pathologies affecting the spine such as Chiari malformation or syringomyelia.
Figures 12.12 and 12.13 present the case of two patients where PC-MRI could quantify CSF flow alterations in the spinal canal. However, these examples do not aim to explain these complex pathologies which, according to previous experience, present large variabilities.
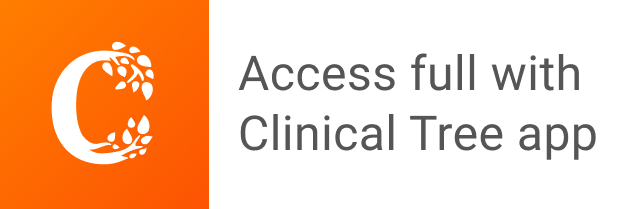