Abstract
This chapter is a brief introduction to neuroimaging, with attention to imaging modalities, indications, and limitations of use. Major modalities for brain and spine imaging will be discussed, with particular attention to types of imaging sequences commonly used in the neurocritical care patient population.
18 Neuroimaging
18.1 Introduction
Neuroimaging plays an essential role in the diagnosis and management of critical neurological disease. Advances in the fields of neurology and neurosurgery have directly paralleled advances in neuroimaging, for which their inventors have won numerous Nobel Prizes since Röntgen developed X-rays. 1 An in-depth discussion of the physics of neuroimaging, interpretation of neuroradiography, and clinical application thereof are beyond the scope of this chapter. The following should serve as a brief introduction to commonly used imaging modalities in the neurocritical care patient.
18.2 Types of Imaging
18.2.1 Brain Imaging
In the modern era, computed tomography (CT) and magnetic resonance imaging (MRI) account for the vast majority of brain imaging studies. This is in large part due to their ease of acquisition, relatively noninvasive nature, multi-planar reconstruction (MPR) of multiple image sets from a single acquisition, and use of advanced imaging techniques. This chapter will also discuss cerebral angiography, which still plays a key role in diagnosis of cerebral vascular diseases, and is essential for neuroendovascular procedures.
18.2.2 Spine Imaging
As with brain imaging, CT and MRI form the core of modern neuroimaging for spinal disorders. They form a direct complement to one another, with CT most useful for imaging bony elements, and MRI most adept at demonstrating neural elements or soft-tissue pathology. The exception to this is CT myelography (CT-M), which uses lumbar puncture to inject iodinated contrast to image the intra-thecal contents. Unlike brain imaging, plain X-rays are used extensively in management of spine disease, particularly in order to demonstrate dynamic changes in spinal alignment to motion or weight-bearing.
18.3 Advantages and Limitations
18.3.1 Brain Imaging
CT
A CT scan produces cross-sectional images (slices) from computer reconstruction of a series of X-rays taken from different angles. In general, findings on CT are described in terms of density: isodense, hypodense, and hyperdense.
Isodense is grey and refers to brain tissue.
Hypodense is dark grey or black and refers to cerebrospinal fluid (CSF) (dark grey) or air (black).
Hyperdense is white and refers to bone, blood, or calcium.
The X-rays are absorbed by tissues depending on density, which is translated to different intensities on a grey scale. The scale of absorption is referred to in terms of Hounsfield units (HU) and ranges from +1,000 (bone) to –1,000 (air) (Table 18‑2). The higher the number the brighter it is on imaging and likewise the lower the number the darker it is. 17
Tissue/Substance | Hounsfield units |
Air | −1,000 |
Fat | −30 to −70 |
Water | 0 |
Muscle/Soft tissue | 20 to 40 |
Brain white matter | 25 |
Brain grey matter | 35 |
Intracranial hemorrhage | 60 to 100 |
Punctate calcification | 30 to 500 |
Iodinated contrast | 100 to 600 |
Bone or metal | +1,000 |
Note: Approximate values. May vary according to source. |
CT has significant advantages that often make it the initial study for patients with suspected intracranial pathology. The ease of acquisition makes it the ideal study for emergent or repeat brain imaging in the acute inpatient setting. 2 Routine head CT imaging (HCT) produces 5 mm thick axial sections, although multi-detector scanners can now generate submillimeter thickness images which accommodate MPR with minimal interpolation artifact. Routine HCT is full of clinically relevant information, such as the presence of brain edema or herniation, ventricular configuration, ischemia, and osseous imaging of the skull and sinuses (Fig. 18‑1). Generally speaking, it is the study of choice for intracranial hemorrhage for several reasons. First, the speed of acquisition allows interventional decisions to be made rapidly. Also, the evolution of blood products from the acute to subacute to chronic phase is much more straightforward than on MRI. Finally, the advent of portable CT scanners has meant that all but the most extremely unstable critical care patients can undergo HCT imaging as clinically indicated. 3

However, CT does have several limitations. As with all X-ray-based imaging technologies, CT requires exposure to ionizing radiation, which is of particular concern with pediatric patients. HCT is also significantly limited in its ability to demonstrate parenchymal changes. Contrast-enhanced HCT can help improve tissue contrast for certain disease states, but does require iodinated contrast administration, with its attendant renal and allergic risks. The major classes of image artifacts encountered in CT imaging include motion (though less so than MRI), streak artifact from metal in the image field, and beam hardening artifact (which limits the utility of HCT in imaging the posterior fossa). 4
Since the time of its creation in the 1970s, CT imaging has continued to evolve and now detailed neurovascular imaging can be provided through angiography, perfusion, venography, and contrast enhancement.
CT Angiography
CT angiography (CTA) has become one of three core imaging techniques for imaging cervical and intracranial vascular disease (see below). High-resolution acquisition can be reconstructed in multiple planes, and can be rendered in three dimensions. In combination with CT perfusion (CTP), CTA is an essential component of neuroimaging in acute brain ischemia when considering endovascular thrombectomy. 5 Acute thrombus is identified as an abrupt cutoff in a vascular tree. CTA approaches the sensitivity and specificity of digital subtraction angiography (DSA) for detection of cerebral aneurysms and other intra-cranial vascular lesions, although without the benefit of dynamic imaging of cerebral blood flow. The presence of vascular intervention devices, such as aneurysm clips, coils, or stents, results in significant streak artifact that can limit the utility of CTA for long-term follow-up. 6 Poor bolus timing (as with cardiomyopathy) can result in poor arterial opacification or venous contamination.
CT Venography
CT venography (CTV) is a useful and rapid imaging technique used to diagnose cerebral venous thrombosis. The CTV is reported to have 95% sensitivity with multi-planar reformatting compared to traditional DSA. 19 It can provide detailed anatomy of intracranial venous system, both the deep and superficial veins. That being said, it is common to have normal variants of the venous system and should not be mistaken for pathologic event. 18 It carries the same limitation as any study that requires contrast and should be used with caution in pregnancy.
CT Perfusion
Described first by Axel in 1980 22 , perfusion imaging of the brain has taken on a core role in the acute management of ischemic stroke. At a basic level, perfusion imaging relies on dynamic imaging of a contrast bolus through a volume of tissue. Parenchymal blood flow parameters can be mapped on a voxel-by-voxel basis, and reconstruction of perfusion maps allows for identification of cerebral blood flow (CBF), mean transit time (MTT), and cerebral blood volume (CBV), which are related by the equation:
Clinical application of this imaging technique allows for delineation of ischemic/oligemic versus infarcted cerebral tissue. Regions of ischemia significant enough to produce cell death (less than 8–10 mL/100 cc tissue/minute) will have matched regions of decreased CBF and CBV (Table 18‑3). However, intermediate ranges of ischemia or oligemia can result in impaired neuronal function without cell death, resulting in potentially reversible clinical symptoms. This is seen as regions of decreased CBF but with preserved CBV, which receive enough collateral flow to preserve neuronal integrity for a period of time, known as the ischemic penumbra, which can potentially be salvaged with thrombolytic or mechanical thrombectomy therapies (Fig. 18‑2). 7 CT-based perfusion imaging is, generally speaking, more reliable than MR perfusion (MRP) due to the fixed relationship between iodinated contrast concentration and density, unlike the nonlinear relationship between Gd-contrast and the T2* signal which forms the basis for MRP.

Contrast
While brain MRI is the imaging modality of choice for evaluation of intracranial pathology, contrast-enhanced head CT still has some utility. Most commonly, it is utilized for patients with non-MRI compatible metallic or implantable hardware. Additionally, in some cases, contrast imaging is preferred or required. Patients with renal failure are unable to receive gadolinium (Gd)-based contrast due to the risk of development of nephrogenic systemic fibrosis (NSF) and CT contrast is iodinated which can be dialyzed.
MRI
MRI is based on the principle in physics of nuclear magnetic resonance. 8 It uses interactions of hydrogen nuclei, radiofrequency energy, and a magnetic field. The majority of images are created from the magnetic properties of the hydrogen nuclei contained with water molecules within tissue, of which the human body is abundant. The exact physics behind the individual sequences are beyond the scope of this chapter.
MRI has far greater brain tissue contrast compared to CT. This is particularly true for new higher field strength magnets (3 Tesla or greater), although with notable limitations for certain types of artifacts. 9 MR-based imaging is also capable of a wide array of advanced imaging techniques such as diffusion imaging or spectroscopy, and does not use ionizing radiation. Conversely, MRI is significantly more susceptible to motion artifact than CT, requires longer image acquisition times, is significantly more expensive to install and maintain, and requires safety protocols for patients and equipment in the magnetic environment. In comparison to CT, findings are referred to in terms of intensity: hypointense, hyperintense, and isointense. There are various sequences that are completed with routine MRI which are noted within Table 18‑4.
Diffusion
Diffusion-weighted imaging (DWI) is currently the imaging modality of choice for the evaluation of cerebral infarction. DWI utilizes motion of water molecules for tissue contrast. Normal brain tissue has relatively low diffusion restriction, as the majority of water is contained in the interstitial space. However, in the setting of stroke, Na2+/K+ pump failure results in loss of cellular homeostasis, sodium influx, and net inflow of water into the intracellular space. This results in an extremely conspicuous hyperintense signal in the region of infarction referred to as diffusion restriction. A similar effect is seen with cerebral abscesses and epidermoid cysts, both of which show diffusion restriction on MRI. Due to short acquisition times, DWI can be performed quickly and is relatively resistant to motion artifact. However, DWI can be significantly limited by susceptibility artifact, particularly near the skull base due to air–tissue interface, in the region of blood/blood products or from metal-containing hardware. Diffusion imaging can also be complicated by the presence of underlying edema, which independently shows up hyperintense on DWI with the absence of true diffusion restriction. This is called T2 shine-through which refers to a hyperintense signal on DWI image without restricted diffusion but due to a high T2 signal which “shines through.” 10 Use of apparent diffusion coefficient (ADC) maps can help delineate the difference between true diffusion restriction and T2 shine-through, with dark regions on ADC correlating with diffusion restriction. ADC changes also are the earliest identifiable features on MRI in the setting of acute stroke.
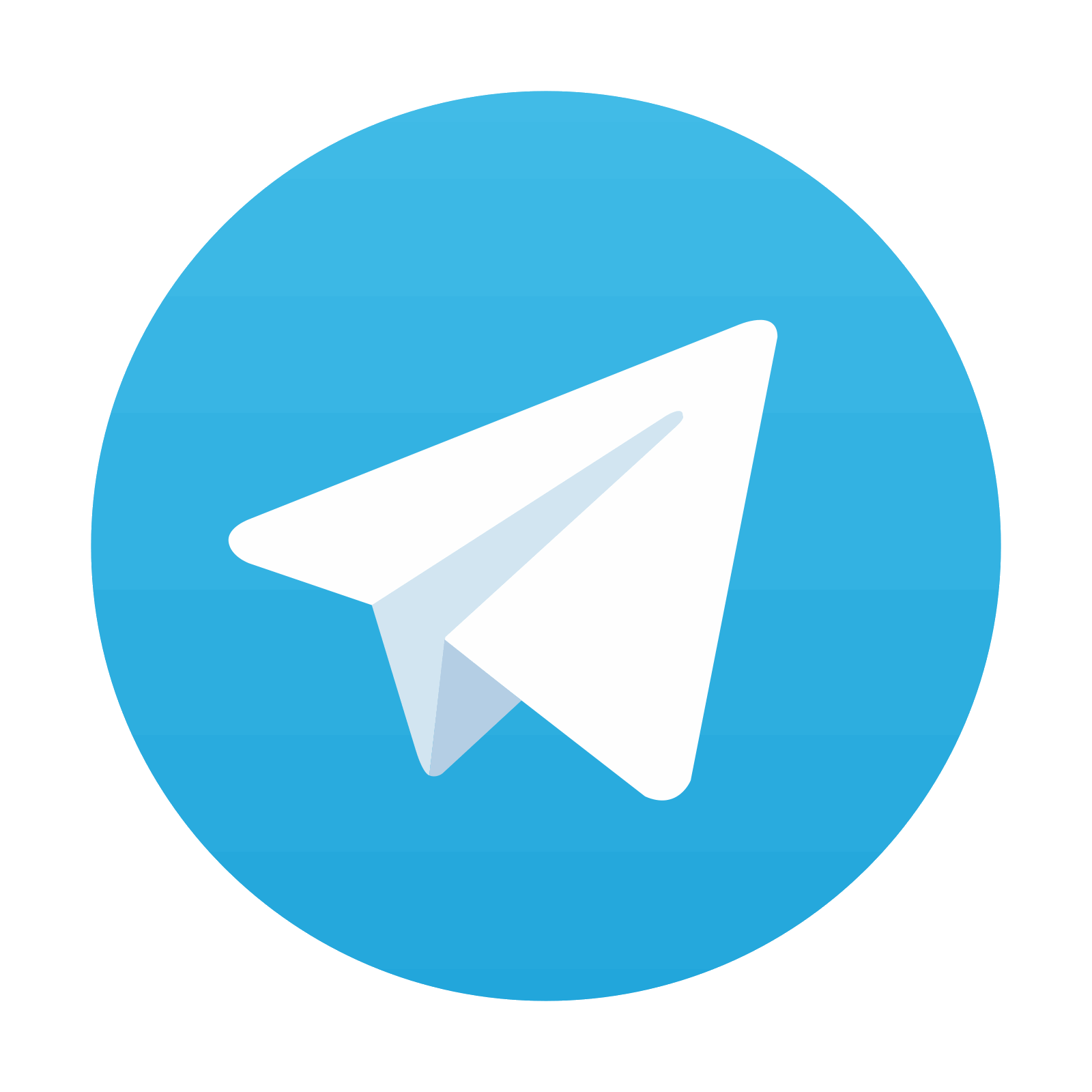
Stay updated, free articles. Join our Telegram channel
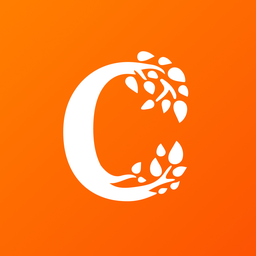
Full access? Get Clinical Tree
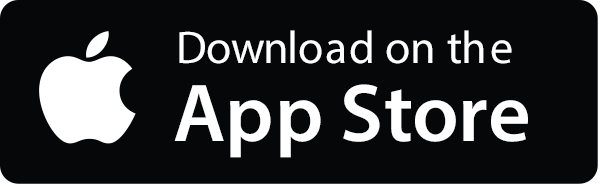
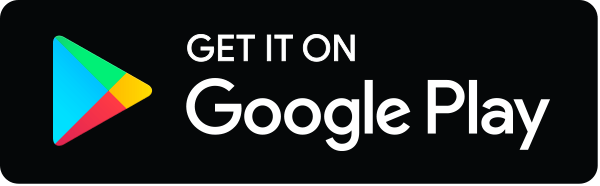
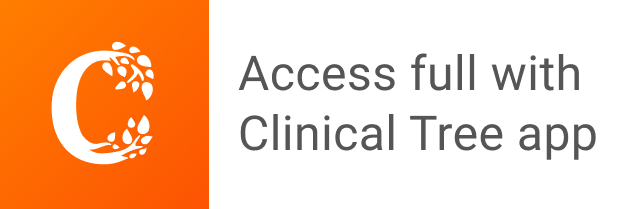