20 Craniocervical and Upper Cervical Constructs
The anatomical and associated clinical complexities of the upper cervical spine and craniocervical junction pose significant challenges for the managing surgeon 1 , 2 : (1) the high risk for treatment failures, (2) the significant multiplanar forces affecting this region, and (3) the difficulties associated with attaining a solid fusion in the upper cervical spine–occiput (craniocervical junction) region. This chapter focuses on the “old,” and merges into the “new,” regarding implant construct design and implementation.
It goes without saying that trauma to the upper cervical spinal cord and region of the foramen magnum—whether surgical in nature (iatrogenic), the result of spinal instability, secondary to extrinsic neural compression, or the result of trauma—is associated with significant morbidity and mortality, as well as with significant clinical confusion. 3 For example, a simple procedure, such as the passage of transarticular screws, places pharyngeal soft tissues and the vertebral arteries, as well as the spinal cord, at risk. Similarly, occipitocervical fusion is associated with its own set of significant complications. Regardless of the risk of surgery, however, the best predictors of outcome are preoperative clinical metrics. For example, the best predictors of outcome following occipitoatlantal disclocation include severe brain and upper cervical spine injuries at presentation. 4
The aforementioned challenges are, at least in part, related to the significant multiplanar forces that impinge upon the craniocervical region, and the sometimes extreme means required to resist them. Axial, angular, translational, and combinations of force vectors can result in a variety of craniocervical injuries. The actual injury to bony and soft tissue structures depends on the orientation of the injury force vector, its magnitude, and the intrinsic strength of the multitude of involved spinal elements (see Chapter 6 and Fig. 20.1 ).

Like Chapter 16, this chapter focuses on principles. Therefore, a knowledge of historical techniques and applications is relevant. They are presented here for illustrative purposes. The intent is not to encourage their use, but to use the mechanics involved in their application as a tool for learning.
Solid arthrodesis is, relatively speaking, difficult to achieve in the craniocervical region. This is primarily related to two factors: (1) the multiple motion orientations that must be restricted by the implant and (2) the geometry and characteristics of the ligamentous soft tissue and bone in this region. 5 Flexion, extension, and rotation (about the long axis of the spine) are extensive in the upper cervical spine (Table 20.1 ). Simply restricting flexion and extension may not be enough. Rotation may interfere with the fusion process enough to render it unsuccessful. For example, dorsal C1–C2 wire fixation strategies can resist flexion and extension relatively effectively. However, they resist rotation and translation poorly (Fig. 20.2a–c). This may result in failure of arthrodesis. In addition, rotation may adversely affect rates of craniocervical arthrodesis. Both may be aided by the use of transarticular screws, which resists C1–C2 rotation very effectively (Fig. 20.2d). The flexion and extension resistance of translational screws is enhanced by the addition of a wire fixation component (Fig. 20.2e, f).

Joint | Motion | Range of motion (degrees) |
Occiput–C1 | Combined flexion and extension | 25 |
Lateral bending (unilateral) | 5 | |
Axial rotation (unilateral) | 5 | |
C1–C2 | Combined flexion and extension | 20 |
Lateral bending (unilateral) | 5 | |
Axial rotation | 40 |
The geometry and characteristics of the native bone of the craniocervical region impose additional challenges, particularly in patients with congenital and genetic disorders. 6 , 7 Bone graft recipient beds are often separated significantly (e.g., occiput–C1 and C1–C2). These gaps create significant barriers to arthrodesis. The compact (cortical) nature of the bone of the occiput (membranous bone) also makes early union, and therefore ultimate arthrodesis, less likely (Fig. 20.3). An appreciation of the size and caliber of implant anchor sites of affixation is critical, particularly when screw fixation is being considered in children. 8

Finally, the integrity of a dorsal fusion is threatened by flexion moments. These place bone grafts under tension and create gaps between fusion beds and bone graft. Older techniques (Fig. 20.4) are particularly prone to this.

20.1 Surgical Strategies: Overview
In general, the optimal arthrodesis uses both cortical and cancellous bone. The cortical bone provides early structural integrity. This is most important with respect to ventral load-bearing fusion. The cancellous bone promotes early fusion by increasing the contact surface area and by facilitating early vascularization. Early structural integrity may be important with regard to dorsal fusions, so that the cortical portion of the bone graft is clinically significant (Fig. 20.5). A spinal implant can provide the early stability that the cortical portions of bone grafts would normally provide, thus making the cortical portion less necessary. In this latter situation, in which the implant provides the initial structural integrity, morselized bone may be adequate and in fact may be optimal (Fig. 20.6).


According to Wolff’s law and its corollaries, bone heals best under compression. Compression creates a negative charge on the surface of bone, which is conducive to healing via the stimulation of osteoblast activity. Therefore, compression between healing bone segments should be sought when possible. This can be achieved via the lag screw effect, such as that employed with odontoid screw fixation (Fig. 20.7), or via axial load bearing and compression of the bony fusion with the application of compressive loads—for example, dorsal craniocervical constructs in a compression (tension-band fixation) mode (Fig. 20.8).


Care must be taken to perform the individual steps of a complex operation in the appropriate order. For example, if C1–C2 transarticular screws are placed before a dorsal C1–C2 wire tightening, the dorsal bone graft may initially be placed in compression. However, as the wires relax or “cut” into bone, the rigid nature of the transarticular screws and their fixation may cause the C1 and C2 laminae to resume their prewired (relaxed) position. This can result in tension and the formation of a gap at the dorsal bone graft site, which in turn may result in a failed arthrodesis (Fig. 20.9).

Craniocervical surgery is not without complications, particularly in the aged and the otherwise medically compromised. Therefore, nonoperative strategies should always be carefully considered during the decision-making process. 9 – 11 Some complications are not immediately obvious. For example, subaxial cervical alignment and spinal balance can be affected by the C1–C2 fixation angle. 12 , 13 A hyperlordotic or hyperkyphotic C1–C2 fixation angle results in a relative subaxial kyphosis or hyperlordosis, respectively (Fig. 20.10). Such alignment derangements can result in degenerative changes, as well. 13 Of note, Mukai et al observed no consistent relationship between C1–C2 fixation angle and subaxial spine deformation in patients with rheumatoid arthritis. 14 Such biomechanical factors, nevertheless, should be taken into consideration in the planning and performance of such operations.

The correction of upper cervical and skull base deformity and other aggressive pathologies (e.g., neoplasm) can be addressed with a variety of strategies. Most, but not all, involve fixation to the occiput. 15 – 17 Goel has managed such deformities with an atlantoaxial joint distraction technique. 18 The biomechanics of this technique have been studied and are favorable. 19
Surgical strategies, from a biomechanical perspective, can be studied in the laboratory. A comparison of techniques is thus made possible. 11 , 20 – 28
20.2 Ventral Constructs
Ventral craniocervical and upper cervical constructs include odontoid screw fixation, ventral short fusion strategies, plate fixation, and transarticular screw fixation. Ventral surgical strategies are complicated by location because oral or high extrapharyngeal surgical approaches are required to access the pathology. Although there is an obvious advantage to decompression and stabilization through the same approach, biomechanical and anatomical restrictions often limit the utility of such a strategy (Fig. 20.11).

20.2.1 Odontoid Screw Fixation
Odontoid screw fixation is a viable means of managing acute type II odontoid (dens) fractures (of Anderson and D’Alonzo). It is by far the most common and popular ventral craniocervical and upper cervical spine stabilization technique. 29 It takes advantage of the lag screw effect, which can be achieved by overdrilling the proximal bone fragment (C2 body) and using a fully threaded screw, or by using a lag screw (Fig. 20.12). Compression of the separated dens into the C2 body can thus be achieved. Care must be taken to avoid three situations with this technique: (1) Old fractures in which a fibrous nonunion has developed are associated with suboptimal arthrodesis rates; (2) diagonal fractures predispose to angulation and translation of the bone fragments during compression (Fig. 20.13); and (3) significantly comminuted fractures do not allow a proper compression effect. Their comminuted nature, combined with motion, increases the incidence of arthrodesis failure (Fig. 20.14).



There is controversy over the virtues of using one or two screws for odontoid screw fixation. 30 Proponents of using two screws cite a rotation-limiting effect that the moment arm created by the two screws provides. There are several problems associated with this rationale for the use of two screws. First, rotation of the dens on the body of C2 is likely of minimal concern in most clinical situations. The irregular surfaces of contact limit rotation via compression created by the lag effect. Second, the two screws must be placed close together. Any effect to prevent rotation is created by the bending moment related to the two screws. This, in turn, is related to the applied moment arm, which depends on the separation of (distance between) the screws. Because the screws are juxtaposed, this effect is essentially nil (Fig. 20.15). Third, the use of two screws potentiates a less than optimal placement for one or both screws (Fig. 20.16) and also results in the implant occupying twice the volume of bone, thus further decreasing fusion potential and ultimate construct strength. This is particularly true as the screws become fatigued following repetitive loading (cycling).


20.2.2 Ventral Strut Fusion Strategies
Ventral craniocervical and upper cervical interbody and strut grafting present unique advantages and disadvantages. 31 The advantages are obvious. The opportunity to decompress and fuse and/or apply an implant through a single approach is very appealing. The main disadvantage, however, is that this is rarely feasible. Problems with infection and the logistics of surgery are also major drawbacks.
From a biomechanical perspective, ventral struts are associated with significant obstacles in the craniocervical region. They are very ineffective at resisting rotation (Fig. 20.17a), flexion–extension (Fig. 20.17b), and translation (Fig. 20.17c). They interface with bone of widely disparate densities and moduli of elasticities. The relatively soft bone of the vertebral bodies of the axis and subaxial cervical spine contrasts significantly with the relatively hard bone of the clivus. Therefore, the selection of a bone graft strut is complex. Should a relatively soft tricortical iliac crest, which has approximately the same density and cortical-to-cancellous ratio as the axis and subaxial vertebral bodies, be used, or should a fibula, which more closely approximates the density of the clivus, be used? Other issues that must be addressed include the following: (1) the extent of dorsal element integrity and its ability to resist excessive motion, such as translation; (2) the risk for dorsal graft migration with neural element impingement; and (3) infection.

20.2.3 Ventral Plate Fixation
A number of ventral upper cervical plating techniques have been devised. A rudimentary technique is portrayed in Fig. 20.18a. Such techniques are complicated by two predominant clinical factors: (1) the relatively weak screw fixation points and (2) the risk for infection secondary to transoral contamination. The former have been addressed with a variety of strategies (Fig. 20.18b–e). Each provides advantages and disadvantages from a biomechanical perspective. 11 The subarticular atlantoaxial locking plate (SAALP) appears to provide an optimal biomechanical advantage. 11 However, the relatively weak screw fixation and risks for contamination and infection limit its use. 32 Of further note in this regard is that transoral upper cervical plate fixation is less stable than combined ventral and dorsal reconstruction procedures. This limits its use as a “free-standing” stabilization strategy. 33 , 34

C1–C2 ventral cervical plates dictate the screw entry point, which may provide suboptimal fixation. Hence, a solid knowledge of the anatomical relationships between the fixator and the intrinsic spine anatomy on a case-by case basis is essential. This has been corroborated by anatomical data. 35
20.2.4 Ventral Transarticular Screw Fixation
Ventral transarticular screw fixation strategies (Fig. 20.18f) are limited predominantly by the risk associated with screw violation of adjacent vascular and neural structures, as well as the risk for infection. Their use, therefore, is significantly restricted. 36
20.3 Dorsal Constructs
Dorsal craniocervical and upper cervical constructs include occipitocervical fixation, C1–C2 fixation, and more caudal extensions of both. Wires, screws, clamps, and hooks may be used to anchor to bone.
Dorsal craniocervical and upper cervical constructs, in general, pose fewer problems and are associated with fewer or less significant complications than their ventral counterparts. 37 They provide much greater stabilization potential, 38 as well, particularly if three points of fixation are used. 39 Because the strategies used with dorsal techniques are very different from those used ventrally, and because dorsal strategies are much more familiar to spine surgeons, a different approach to their description than that used for the ventral approaches is undertaken here. Fixation strategies and length of construct considerations are specifically addressed. Wire, cable, hook, clamp, screw, and button fixation are addressed separately, as is length of fixation.
20.3.1 Wire and Cable Fixation
Wire and cable fixation to the occipital bone, laminae, and spinous processes is a “tried and true” method of bone anchoring. In general, cables are stronger and more resistant to notching and fatigue failure than wires. Stainless steel is stronger than titanium. Polyethylene cables have as much tensile strength as stainless steel cables but are much more susceptible to stretching or creep. 40 Some surgical techniques are better than others. Obviously, these are, at least in part, dictated by circumstance. For example, the Gallie technique is relatively poor at rotation, flexion, extension, and lateral bending resistance, compared with the Brooks technique, clamps, and transarticular screw fixation. 41
Wires and cables tend to loosen via “cheese cutting” through bone. Significant complications can subsequently arise. 42 This effect is due predominantly to the relatively small surface area of contact between the bone and the wire or cable, which can be increased by using parallel double wires or cables. Crossing or overlapping the wires negates the double-wire surface area of contact strategy (Fig. 20.19). Care must be taken to prepare occipital bone sites meticulously so that a perpendicular (or lesser) angle of exposure to bone is achieved. A greater angle of exposure enhances the chances and extent of “cheese cutting” and so loosening (Fig. 20.20).


Spinous process wiring is not as strong as, but possibly safer than, sublaminar wiring. However, care must be taken to prevent wire passage through the canal (Fig. 20.21).

Gaps between a rod and bone are not desirable. Therefore, careful rod or plate contouring is strongly recommended. Ideally, rods for sublaminar wire or cable attachment should be placed in the trough lateral to existing spinous processes so that gaps between the laminae and rods are eliminated bilaterally (Fig. 20.22). Cables significantly increase stiffness. 43 In addition, cables encroach less on the spinal canal than wire during insertion. 43 , 44 Rotation and translation prevention, particularly with short (e.g., occiput–C2) constructs, is limited (Fig. 20.23). Nevertheless, cabling techniques may be useful for occipitocervical fixation. 45


Wire may also be used for fixation with polymethylmethacrylate. This increases the failure load. 46
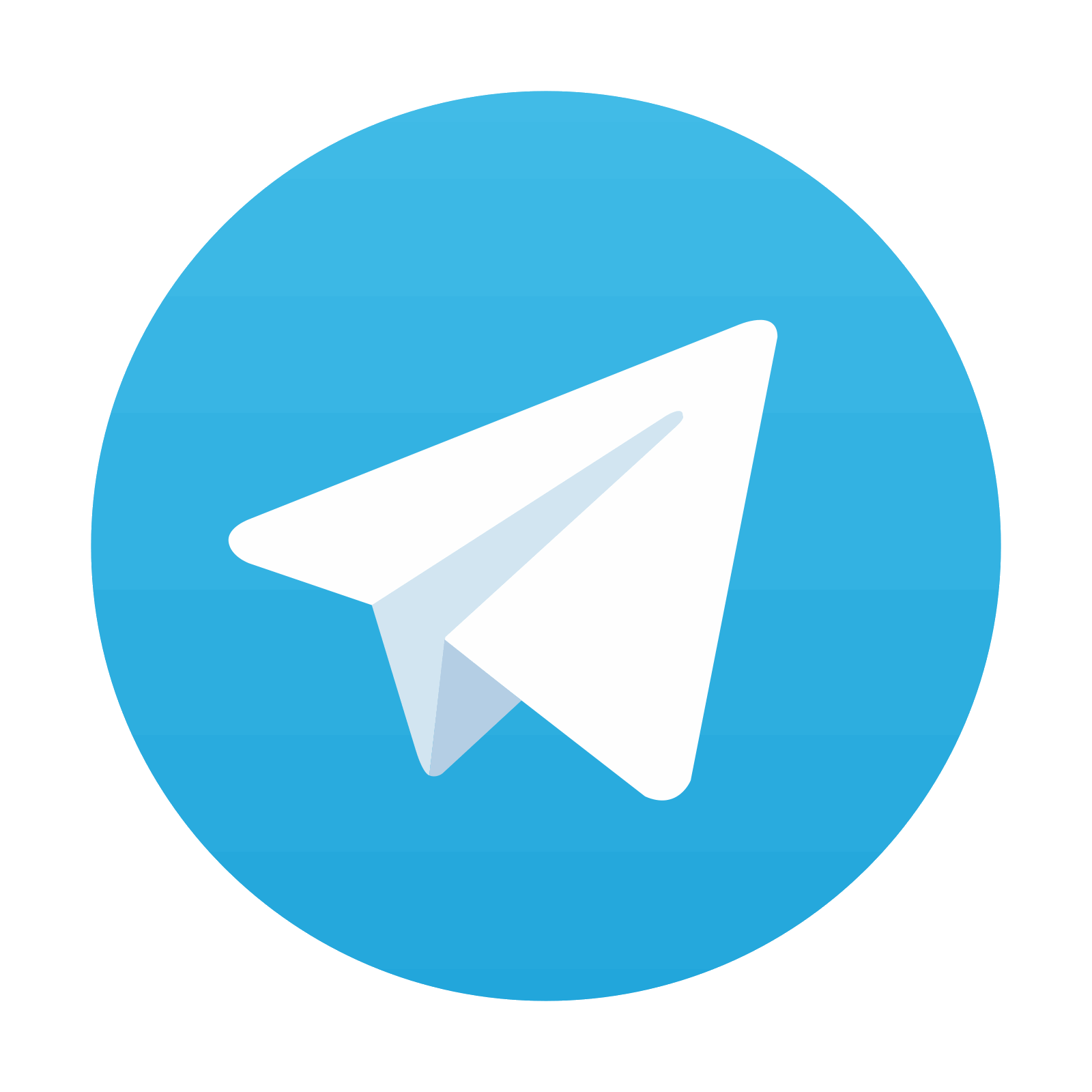
Stay updated, free articles. Join our Telegram channel
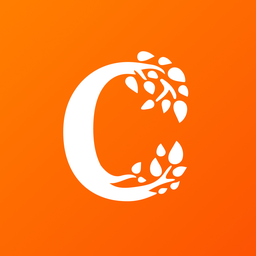
Full access? Get Clinical Tree
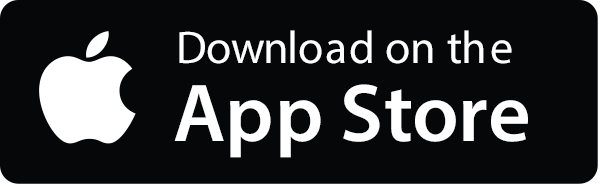
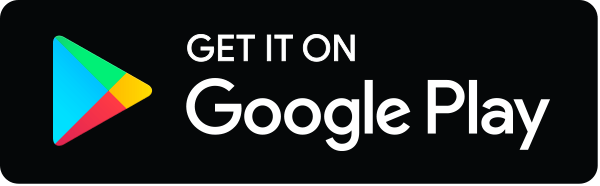
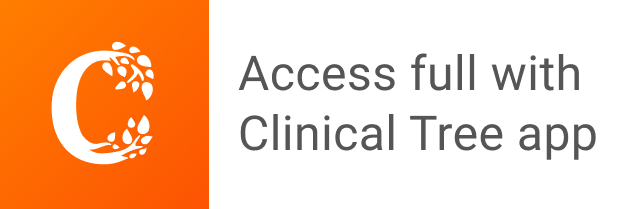