21 Cerebral Microdialysis in the Neurosurgical Intensive Care Unit
Abstract
While monitoring intracranial pressure is meaningful for a mechanical understanding of brain pathology, it does not provide a comprehensive picture of alternations in the brain from disease and trauma; understanding the chemical changes in the brain, as reflected in the cerebrospinal fluid and brain interstitial fluids, provides an additional (biochemical) window into brain damage and dysfunction. Cerebral microdialysis provides a nuanced approach that is relatively new to the neurosurgical intensive care unit, and not yet universally utilized due to its labor intensiveness in data collection and analysis; it is the next frontier in brain monitoring.
Case Presentation
A 20-year-old right-handed man was admitted to the trauma center after sustaining a traumatic brain injury in a motor vehicle collision. His best examination after resuscitation was E = 1, V= 1, M = 3, with a Glasgow Coma Scale score of 5. He had bilaterally reactive pupils. His computed tomographic scan of the head demonstrated right-sided subfrontal contusions with some surrounding edema with open perimesencephalic cisterns. A ventriculostomy was placed with initial intracranial pressure = 25 mm Hg. This decreased to 15–18 mm Hg with sedation, elevation of the head of the bed, and hypertonic saline. A Licox brain oxygenation monitor and cerebral microdialysis catheter were placed via a double-lumen bolt.
See end of chapter for Case Management.
21.1 Introduction
The cerebrospinal fluid (CSF) traditionally has been felt to be reflective of the overall biochemistry of the brain. The bulk flow of CSF through the brain, however, may follow different pathways that may not all lead to the cerebral ventricular system. The recent finding of a lymphatic system in the brain has raised questions about the relationship between the brain interstitial fluid and composition in comparison to CSF. 1 , 2 , 3 Indeed, it is quite clear that the ventricular CSF and interstitial glucose, lactate, pyruvate, and amyloid beta levels are different. 4 , 5 As such, sampling the CSF for metabolic or proteinomic biomarkers of traumatic brain injury (TBI) or cerebrovascular accident may provide information that is different from the sampling of the brain interstitial fluid. Cerebral microdialysis allows the sampling of cerebral interstitial fluid and is reflective of the local milieu around the catheters. 6 , 7
Cerebral microdialysis was first developed as an experimental tool. Much of the early work was pioneered by Urben Ungerstedt, who was able to collect small molecules from the brain interstitium for analysis. 8 , 9 The early work in the laboratory used the principles of hemodialysis and developed techniques to miniaturize the technology to enable the placement of the microdialysis membranes safely into the brain. 10 , 11 The microdialysis catheters have evolved and have been developed for human use. This catheter has an inner tube and an outer tube that has a semipermeable membrane at its tip. Through the inner tube is pumped the perfusate (usually artificial CSF). This perfusate exits the tip of the inner tube and goes into the outer tube. As the perfusate flows through the region of the semipermeable membrane, exchange occurs with the brain interstitium that is in contact with the semipermeable membrane. Molecules that are in higher concentration in the brain flow into the 1-cm tip of the microdialysis catheter that has the semipermeable outer membrane. The dialysate that exits is collected and analyzed. 5 , 12 This is depicted in ► Fig. 21.1. Early studies in humans analyzed the dialysate using fractionation by high-performance liquid chromatography. 13 , 14 , 15

Cerebral microdialysis has become less of a research tool and has become a routine clinical measurement in many centers after the advent of the automated cerebral microdialysis analyzers that analyze the microdialysate for specific substances using a chemical analysis paradigm. These were approved by the U.S. Food and Drug Administration in 2005 for clinical use. 12 , 16 The microdialysis analyzer is now in its second generation and is the Iscus-Flex model (M Dialysis). In principle, all of the compounds that are of a higher concentration in the brain interstitium than in the perfusate may be collected and analyzed. This applies to small chemical molecules, such as glucose, as well as proteins. However, there are several considerations based on the molecular weight cutoff of the membrane. The standard microdialysis catheter available in the United States has a 20 kDa cutoff (no. 70 Microdialysis Catheter, M Dialysis). The most common analytes are glutamate, aspartate, glycerol, glucose, lactate, and pyruvate. These are also the ones that are FDA approved for analysis by the Iscus-Flex. The importance of these particular analytes will be discussed subsequently. Beta amyloid may also be isolated in the dialysate as its molecular weight is about 4.5 kDa for the ABeta 40 and ABeta 42 peptides. In contrast, the tau proteins are 49–67 kDa and may be isolated if one uses the microdialysis catheter with a 100 kDa cutoff. These catheters are currently not available except under a research protocol in the United States. 4
The size of the molecule of interest as well as its charge and lipid solubility affects the ability to collect it. The ability to cross the dialysis membrane will affect the amount that is recoverable. The rate of perfusion of the dialysate will also determine the recovery of the substance. The slower the perfusion, the greater the recovery. 17 For the major biomolecules of interest that were noted above, the standard perfusion rate employed is 0.3 µL/min. This allows for adequate dialysate (18 µL) each hour to be sampled by the Iscus-Flex machine. This slow and constant perfusion is provided by a microinfusion pump. 12
21.2 The Rationale for the Study of Cerebral Metabolites
There was a great deal of interest directed at studying the excitatory amino acids (glutamate, aspartate) with the use of cerebral microdialysis. Glutamate and aspartate levels were found to be elevated after TBI and after subarachnoid hemorrhage (SAH), indicative of tissue damage. In fact, the 2004 international consensus statement on cerebral microdialysis included recommendations to follow these analytes as indicators of brain injury and ischemia. 18 , 19 Glutamate levels can be followed as a marker of ischemia and also as a marker of the severity of the brain injury. Chamoun et al, in 2010, found that there were two patterns of glutamate expression in TBI. One group had the increase in glutamate with a peak at 5 days then a gradual decline. The other group had a constant increase in the glutamate levels. The first group had a lower mortality rate (17.1 vs. 39.6%) and a better 6-month functional outcome (41.2 vs. 20.7%) in comparison to the second group. 20 , 21 The measurement of cerebral glutamate is considered an option in cerebral microdialysis and may be useful in the estimation of prognosis. 22 Glycerol is also considered an option in the 2014 international consensus statement on cerebral microdialysis. This is a marker of cell membrane breakdown; however, it has limited specificity. Furthermore, to date, there has not been the establishment of a relationship between glycerol levels and outcome. 22
Perhaps some of the best studied cerebral analytes are glucose, lactate, and pyruvate. These are very closely linked to each other because of their roles in the Kreb’s cycle. 12 The brain has an extreme thirst for glucose because it is the main energy substrate. Low brain glucose levels (< 0.8 mM) are associated with poor outcomes. These low levels are common in TBI and SAH, largely because these injuries induce a hypermetabolic state. 23 The importance of the maintenance of the cerebral glucose became apparent with the introduction of tight glycemic control in the intensive care units. Although there were better outcomes reported with the use of tight glycemic control, the subset of patients that had worse outcomes were those with brain injuries. Tight glycemic control was defined as systemic glucose of 80–120 mg/dL (4.4–6.7 mmol/L) and intermediate as 121–180 mg/dL (6.8–10.0 mmol/L). Episodes of low cerebral glucose (< 0.7 mmol/L) were more frequent in the tight glycemic control than in the intermediate group (65% vs. 36%, p < 0.01). 24 Further studies have similarly demonstrated poor outcome and increased mortality with low brain glucose. 25 Thus this led to the concept of a more permissive peripheral glucose in patients with TBI or SAH. 12 , 24 The ability to monitor and fine tune the brain glucose by administering exogenous sources of glucose if low levels are detected by cerebral microdialysis has the potential to improve outcome. We routinely use brain glucose levels to guide us in instituting nutrition. Upper limits for brain hyperglycemia have not been determined to date. Clearly brain hypoglycemia is more detrimental than hyperglycemia. 22 , 24
Lactate and pyruvate are metabolites of glucose metabolism and are closely linked with the glucose levels in the brain. If we recall the Kreb’s (citric acid) cycle, glucose is broken down into pyruvate during glycolysis. When oxygen is available, the pyruvate is broken down by the citric acid cycle, generating adenosine triphosphate (ATP). When oxygen is limited, the pyruvate is converted to lactate, still generating ATP but in a more inefficient manner that consumes more glucose. This follows the pathway of anaerobic metabolism, and this is what occurs during ischemic situations. 12 , 26 One of the most robust and dependable markers of cerebral stress is the lactate-pyruvate ratio (LPR). When glucose is limited, the LPR increases. In ischemic/hypoxic situations, LPR also increases. Metabolic stress is generally accepted to be when the LPR > 40, although an LPR of 30–35 is concerning. 27 , 28 Persistently increased LPR is related to long-term frontal lobe atrophy. 28
The common analytes and the normal values are summarized in ► Table 21.1. In summary, glucose and LPR are dependable, and abnormalities are reflective of cerebral ischemia or hypoxia. Common scenarios for this are TBI with increased intracranial pressure (ICP) and decreased cerebral perfusion pressure (CPP). This may also occur with cerebral vasospasm after aneurysmal SAH. The pattern seen in these situations is one of decreasing glucose and increasing LPR. Frequently, this pattern is progressive and may direct the physician to intervene to reverse the trend. For example, if one sees a trend with a decreasing glucose, increasing LPR, and increasing ICP in a patient with TBI, this might prompt early institution of hypertonic saline or early removal of a mass lesion (if it has not been removed yet). Another common example where these parameters can guide the neurosurgeon/neurointensivist is in aneurysmal SAH where the ischemia associated with cerebral vasospasm may be detected before it becomes clinically or radiographically apparent. Early institution of hypertensive therapy or augmentation of CPP with ventricular drainage may attenuate the effects of vasospasm. 19 , 22 , 29
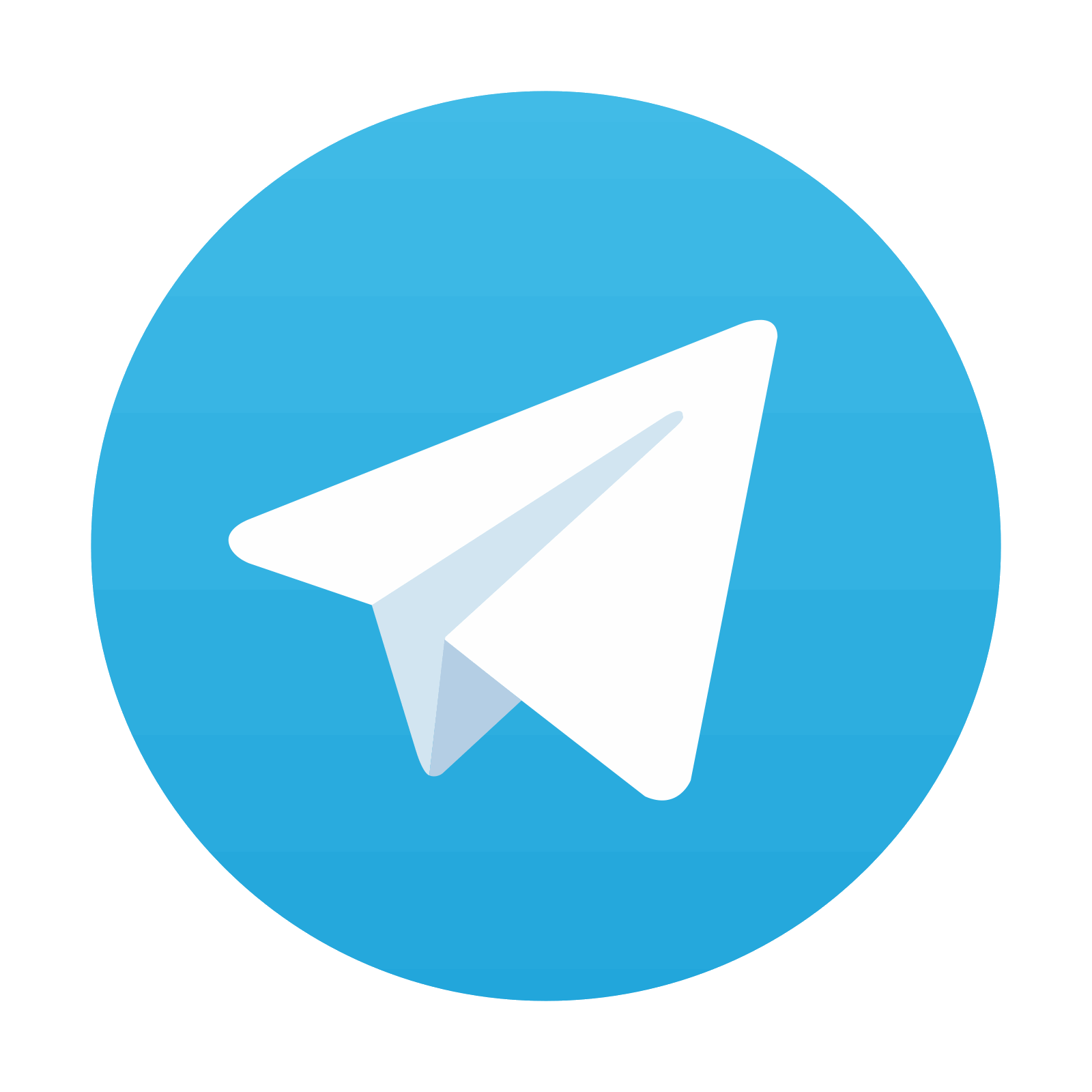
Stay updated, free articles. Join our Telegram channel
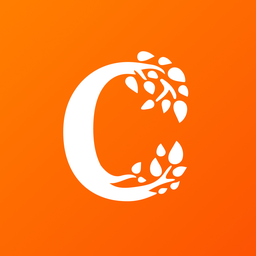
Full access? Get Clinical Tree
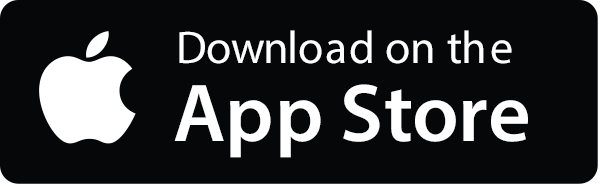
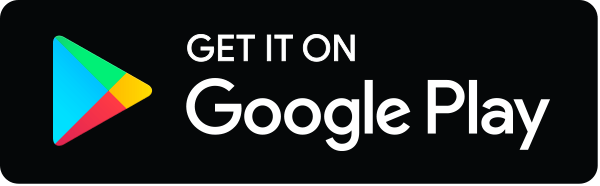