40 New Imaging Techniques in Pediatric Epilepsy Surgery
An essential task in the surgical treatment of epilepsy is the definition of resection margins, which allows the removal of the epileptogenic zone as complete as possible while avoiding neurological deficits. To achieve this goal, the pre-surgical workup includes precise localization of the epileptogenic cortex and of surrounding functionally eloquent cortical areas. Despite all efforts with conventional neurophysiological and brain-imaging techniques, patients who undergo curative epilepsy surgery may receive incomplete focus resections, leaving them with persistent postoperative seizures. The percentage of patients who are not seizure free postoperatively depends on several clinical aspects. Most studies agree that an extratemporal focus and the absence of magnetic resonance imaging (MRI) lesions carries a less favorable outcome, that is, only 30 to 40% are seizure free after surgical intervention.
Up to now, most of these patients have to undergo invasive preoperative electroencephalography (EEG) monitoring during a period of several days or weeks. This monitoring represents a quite demanding situation for the patient and is related to significant medical risks (e.g., bleeding or infection).1 Moreover, intracranial electrodes capture neuronal signals only from a small radius of surrounding tissue,2 which means that the placement of electrodes needs careful elaboration of hypothesis of possible seizure origins. If the intracranial electrodes are not correctly placed, the yield of this exam is reduced. Thus, when patients are supposed to undergo implantation of electrodes for intracranial EEG monitoring, an accurate idea of the cortex to be covered is mandatory.
In addition to the need for exact focus localization, intracranial monitoring may also be required for localization of essential functions, such as motor or language functions, through electrocortical stimulation (ES). ES requires significant time and work-load investment from the medical staff and optimal collaboration from the patient. In addition, the validity of ES alone as a gold standard for functional localization has been questioned, particularly with respect to higher cognitive functions such as language3,4 and are probably partially replaceable by noninvasive techniques. If this lengthy procedure could be abbreviated, this would be most welcome by the patient and the medical team.
Given the significant impact of proper localization of the focus and adjacent eloquent cortex on the surgical results, considerable research has been devoted to sophisticated imaging methods in epileptic patients. Several techniques are addressed elsewhere in this book. Here we concentrate on electric source imaging (ESI) with high-density EEG, on EEG—functional MRI (fMRI), and on the combination of ESI with fMRI by direct recordings of high density EEG in the MRI scanner and simultaneous analysis of the electric and the hemodynamic activity.
Electric Source Imaging
By contrast with fMRI, positron emission tomography (PET) and single photon emission computed tomography (SPECT), ESI directly measures neuronal activity. It is based on the recording of the electric potential field on the scalp using multichannel EEG. The neuronal sources in the brain that produce these scalp potential maps can be estimated using inverse solution algorithms. Despite the nonuniqueness of the inverse problem, sophisticated source and head models now allow a stable and reliable identification of the active neuronal populations in the brain that produces the scalp measurements.5–8 The high temporal resolution (milliseconds) allows tracing the flow of neuronal activity in the whole brain in real time. This high temporal resolution is of fundamental importance in the application to focus localization, where fast propagation of interictal and ictal activity is the rule rather than the exception.9,10 This fast propagation is the reason why ESI and the related technique magnetic source imaging [based on the magnetoencephalogram (MEG)] have particularly attracted epileptologists. MEG is discussed in detail elsewhere in this book, and a recent literature review on its use in presurgical epilepsy is provided in Lau et al.11 MEG relies on the same electro-physiological phenomena (temporally synchronized and spatially aligned postsynaptic potentials) and on the same mathematical underpinnings as ESI. The difference between EEG and MEG has been discussed and debated repeatedly,12–16 but in principle, EEG (when recorded with a sufficient number of electrodes) has higher sensitivity than MEG in the sense that it records neuronal activity independent of the dipole orientation and that it is more susceptive for deeper sources.17 In clinical routine, the practical advantagesof the EEG are obvious, particularly in pediatric and ictal recordings. A very comprehensive review on the yield of ESI in epilepsy can be found in Plummer et al.18 Here we only summarize the basic features and results of ESI.
Number and Positioning of the Electrodes for ESI
The question of the number of electrodes that is needed for proper ESI refers to the question of spatial sampling of the potential field on the scalp and the spatial frequency of this field. Undersampling the field bears the risk of not properly capturing the field extrema and field inversions and thus misinterpreting the sources of this field.6 It has been estimated that electrode spacing of approximately 1 cm is needed to properly measure the potential field.19 Because the electrodes should also cover the low basal temporal and occipital scalp regions, more than 100 electrodes are needed for correct spatial sampling.20 A systematic evaluation of the question of number of electrodes in epileptic patients was performed with a 123-channel ESI recording. The interictal spikes in 14 patients were subsampled to 63 and to 32 channels, and the results were compared with the original 123-channel ESI.21 Localization of the estimated epileptic zone with these three electrode arrays was evaluated with respect to the distance of the resected structure that rendered all patients seizure free. This study showed that localization precision increased significantly with increasing number of electrodes.
However, this study, like many others, used conductivity values of the skull that are probably not adequate. Several recent studies have shown that the skull resistivity is considerably lower than previously assumed22–24 and, therefore, the spatial frequency of the scalp potential field is considerably higher. This lower resistivity is particularly true in newborns and young children, because the resistivity depends on the skull thickness.25,26 Consequently, 256 or more electrodes would be needed in children to minimize spatial sampling errors. Fortunately, this requirement is met by several recent systems, which are commercially available and allow recordings of 256 electrodes or more.27 Fast application of electrode caps with a large number of electrodes is now readily possible, making high-density EEG feasible in clinical routine.28,29
Source Model
EEG source imaging in epilepsy often uses distributed source localization procedures instead of the initially proposed equivalent dipole fitting procedures (see Plummer18 for a review). Distributed source models have the advantages that there is no need for a priori assumption of the number of sources and that they allow an estimation of the extent of the active sources. Distributed source models also better localize propagated activity that often includes multiple simultaneous active sources. Distributed source models estimate the “best” current density distribution in the whole three-dimensional brain space that produced a given recorded scalp potential field.6 The source space is restricted to the gray matter of the cortex obtained from MRI segmentation. Because the problem is highly underdetermined (there are much more solution points than electrodes), additional constraints are needed. Such constraints can be purely mathematical or can incorporate biophysical or physiological knowledge or even incorporate findings from other structural or functional imaging modalities.30 Many advances in source models have been made in recent years. The use of realistic head models and the coregistration of the solutions with the individual three-dimensional brain MRI has ultimately converted the EEG to a functional imaging procedure as capable as other imaging techniques, in keeping with the advantage of directly recording neuronal activity in real time. Examples are shown in Fig. 40.1.
Clinical Applications of ESI
Most of the clinical ESI studies analyzed interictal spike activity. Ray and colleagues31 convincingly showed in simultaneous intracranial and scalp recordings that the analysis of scalp spikes provides more reliable localizing information about the epileptogenic zone than the analysis of ictal activity because of limited propagation of spikes. This good localizing information is, however, only true if the beginning phase of the spike is analyzed and not the peak of the spike, which already reflects propagated activity. Lantz and colleagues10 systematically studied spike propagation in 16 patients with symptomatic focal epilepsy who were all seizure free after surgery. The source analysis of the 128-channel recordings revealed that the best localization was obtained when analyzing the EEG at 50% of the rising phase of the spike. In all cases, the active brain area laid within the resected structure. However, at the spike peak, the source maximum was outside the epileptogenic zone in 5 of the 16 patients.
We evaluated the clinical yield and localization precision in a group of 32 patients with different types of focal epilepsy, all recorded with 128 electrodes.28 A correct localization on a lobar level was found in 93.7% of the cases. In the 24 patients who were operated, the maximal ESI source laid within the resected area in 79%.
Even better accuracy was obtained when using statistical parametric mapping (SPM) or nonparametric mapping applied to the distributed source analysis. The earliest significantly active voxels before the spike peak (compared with a prespike period) highly accurately identified the epileptogenic focus in several studies.32–34 In a pediatric epilepsy surgical cohort, SPM was used to study the yield of ESI from standard clinical EEG (i.e., 19–29 scalp electrodes).34 Interictal recordings of 30 children (13 temporal, 17 extratemporal) were analyzed, and their results were compared with ictal SPECT and interictal PET. Localization of the epileptogenic region agreed with the PET in 82% and with the SPECT in 70%, whereas ESI correctly identified the focus in 90% of the patients. However, the study also showed the limitation of the low number of electrodes, particularly with respect to basal mesial temporal sources, where ESI localized incorrectly in the insula or basal frontal regions in a few patients.

Localization of Eloquent Cortex with ESI
Today, fMRI is the most established tool of noninvasive imaging and is discussed elsewhere in this book. Several investigations showed a good correlation between the distance of the resection margin and the motor area identified by fMRI as determined by the presence or absence of the neurological deficit postoperatively.35 However, despite relatively reliable identification of sensorimotor areas with the fMRI (see Figs. 40.2 and 40.3 ), there are drawbacks that affect its clinical acceptance. Sunaert36 discussed the different reasons: besides a relatively high drop-out rate for technical reasons (e.g., movement artifacts, distortions, system instabilities), the most important problem in patients with brain lesions is the possibility of altered vasoactivity that can lead to neuro-vascular uncoupling and incorrect lack of the BOLD (blood oxygen dependent) activation. Consequently, absence of an fMRI activity in a given area does not necessarily indicate that this region is not implied in the function.
MEG and EEG source imaging can be an alternative to localize functional cortex in the presurgical planning. High-resolution MEG systems have been on the market somewhat longer and have, therefore, been used for this purpose in several studies, particularly for localization of sensory and motor cortex. These studies showed good correlation between sensory and motor evoked fields and ES.37,38 Similar good localization has been achieved with ESI applied to somatosensory evoked potentials.39 A recent comparative study of the yield of EEG and MEG for localizing somatosensory activity in children with focal epilepsies indicated comparable good localization precision in all subjects with structurally normal cortex.40 Interestingly, this study showed that EEG source localization was superior to MEG in patients with central structural lesions, because of more radial orientation of the dipoles in theses cases that were not seen by MEG. The authors concluded that “these findings strongly contradict the view that the EEG is in general less exact or less helpful in mapping cortical activity under clinical condition”40, p 1733. This conclusion was based on a study with 122-channel MEG and only 33-channel EEG (i.e., a setting rather unfavorable for EEG). It may be speculated that ESI is even more precise if more EEG channels are used.41 Because this is now easily obtained in clinical routine,28 high-resolution ESI of somatosensory evoked activity should be considered for noninvasive presurgical functional localization of the somatosensory cortex, particularly in patients with cortical malformations ( Fig. 40.2 ).

The use of MEG or ESI for localization of language cortex is rather sparse. However, there is some evidence that the posterior language cortex is better retrieved with MEG, a region that is inconsistently identified with fMRI.42 More studies are needed to judge the yield of magnetic or electric source imaging in localization of language areas in the individual patient.

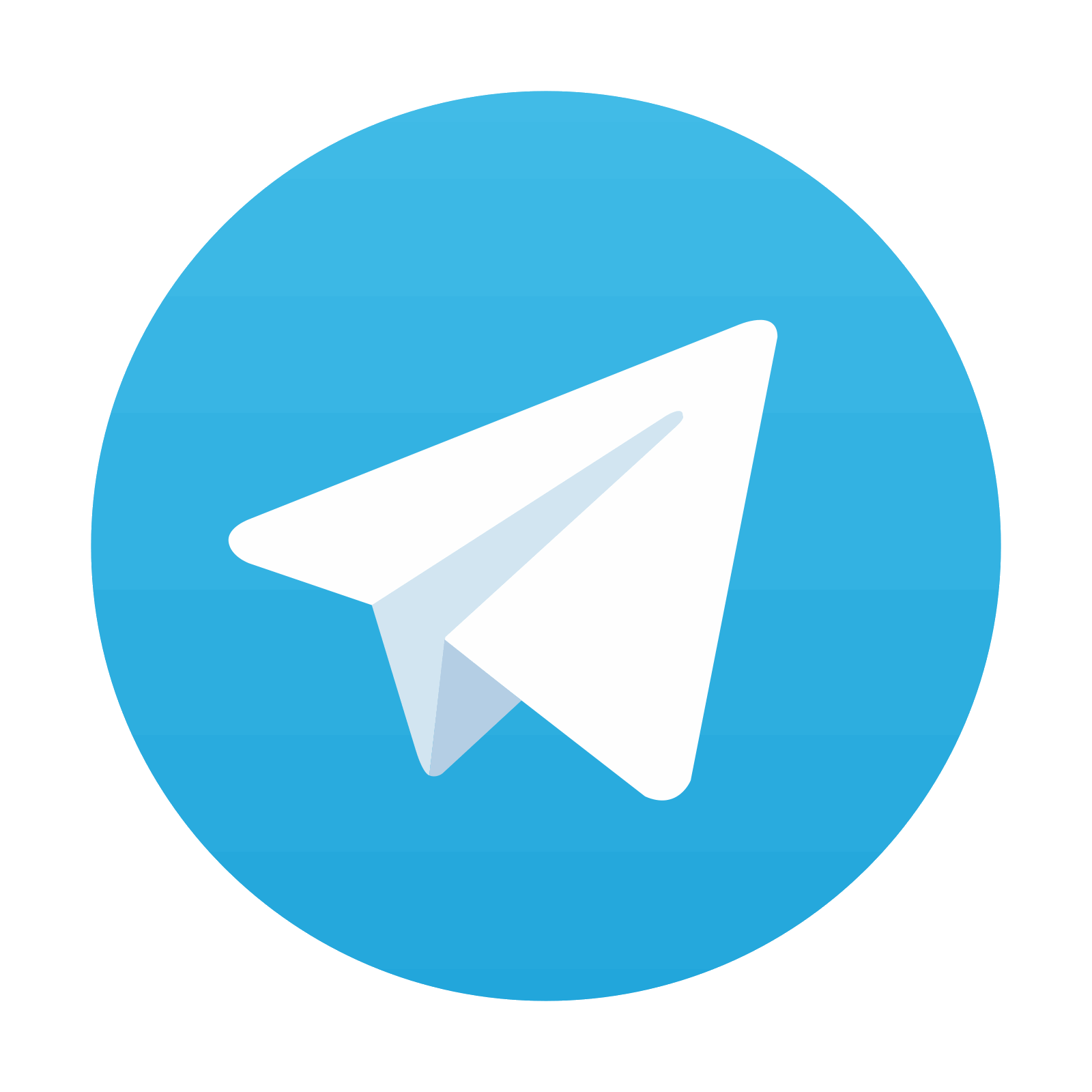
Stay updated, free articles. Join our Telegram channel
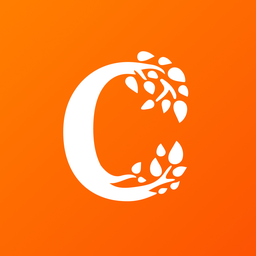
Full access? Get Clinical Tree
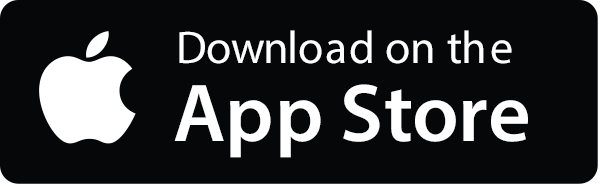
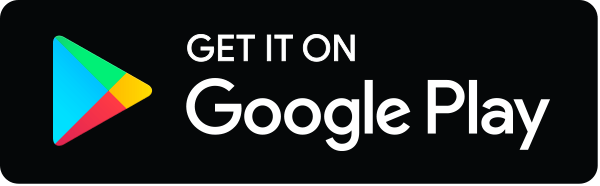
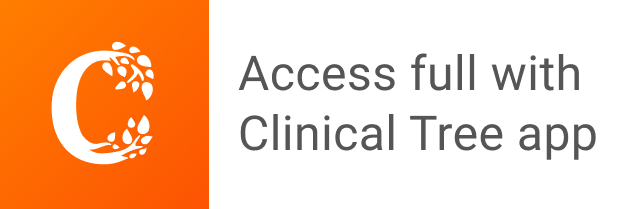