41 Optical Imaging Techniques in Neocortical Epilepsy
Changes in the level of neuronal activity can alter the way in which visible light is absorbed and scattered by brain tissue.1,2 Increases in action potential firing within organized networks of cortical neurons elicit transient optical changes that are localized in the tissue to the areas undergoing increased activity. Because these optical changes arise from the tissue itself and do not involve the application of dyes or contrast-enhancing agents, they are often referred to as intrinsic optical signals (IOSs). IOS imaging involves attaching a sensitive digital camera to an operating microscope, illuminating the cortical surface with light at appropriate wavelengths, and acquiring series of images that can then be processed and analyzed using digital imaging techniques and statistical modeling. This relatively straightforward technique makes it possible to acquire movies showing dynamic patterns of the IOS resulting from action potential firing. IOS-imaging has been used as an experimental technique in the laboratory since the 1980s to study the functional organization of sensory cortex in nonhuman primates.3 It has been most often applied in the laboratory for studying the visual cortex, where it is possible to map functional units such as ocular dominance and orientation selectivity columns.4 In recent years, IOS imaging has been adapted to the operating room where it has been shown to be capable of mapping language, motor, and sensory cortex, as well as the spread of epileptic activity in human subjects.1,5–10
High-resolution IOS imaging has several important limitations. These include its restriction to mapping activity on the exposed cortical surface and its temporal and spatial relationships to neuronal activity, which are still not completely understood. In spite of these limitations, IOS imaging has several major advantages, giving it the potential to be a widely useful tool in the operating room for online mapping of functional and epileptic tissue. Its main advantages are its ability to safely map patterns of cortical activity with micron-level resolution and its relative low cost. Because IOS imaging uses nonionizing light and does not require the use of potentially phototoxic optical dyes, it can safely map the cortex without any possibility of causing tissue damage. In addition, an IOS imaging system is a fraction of the cost and far simpler and more portable than other imaging technologies, such as functional magnetic resonance imaging (fMRI) and positron emission tomography.
A more complete understanding and interpretation of the activity-evoked optical changes in the cortex is required to allow IOS imaging to become a practical and useful clinical tool. An important issue that needs to be better understood is how accurately optical changes at various wavelengths localize normal and epileptic neuronal activity. Resolving this issue requires better elucidating the hemodynamic changes responsible for generating the activity-evoked optical changes in the neocortex. The remainder of this chapter focuses on this issue.
Relationships between the Optical Signal, Cerebral Hemodynamics, and Cortical Activity
Changes in the intrinsic optical properties of cortical tissue are thought to arise from activity-evoked changes in blood volume and blood oxygenation.2 Action potential firing by cortical neurons is known to elicit a transient redistribution of blood within the active tissue, resulting in significant localized increases in blood flow and blood volume.11 Signaling molecules, such as adenosine and nitric oxide, are released by neurons during action potential firing and diffused through the extracellular space to cause nearby pial arterioles to dilate.12 It is known that the smallest pial arterioles with diameters less than 100 μm are the major mediators of this activity-evoked redistribution of blood within the neocortex.13 Poiseuille’s equation predicts that blood flow is related to the fourth-power of vessel diameter,14 and hence small changes in neuronal activity are transformed into large increases in blood flow and volume through dilation of the pial arterioles, whose diameters can increase by 30% above their resting baseline size. The dramatic increase in flow velocity means that the transit time of hemoglobin molecules within the vicinity of the active tissue is significantly reduced, resulting in an increase in the amount of oxygenated hemoglobin in that portion of the venous network receiving the blood from the active tissue.11 Blood oxygen dependent (BOLD)-fMRI, for example, relies on the detection of this activity-evoked increase in oxyhemoglobin (OxyHb).
IOS imaging takes advantage of the fact that OxyHb and de-oxyhemoglobin (Hb) absorb light throughout the visible spectrum differently from each other ( Fig. 41.1A ). Their absorption spectra show that at certain wavelengths, such as 535 nm (green light) and 800 nm (near-infrared light), OxyHb and Hb both absorb exactly the same amount of light, and hence optical imaging at these wavelengths would be insensitive to changes in the oxygen content of blood. By contrast, optical imaging at 660 nm (red light) would be expected to be highly sensitive to changes on blood oxygenation because OxyHb and Hb are maximally distinguishable at this wavelength. Several predictions follow from the facts described in the preceding section. First, it would be expected that during activation, the cortex would absorb more light when illuminated with 535 nm light (i.e., become darker). This follows from the fact that dilating microscopic pial arterioles result in an increase in the number of light-absorbing hemoglobin molecules within a volume of tissue, and so more light would be expected to be absorbed at 535 nm, a wavelength that is insensitive to changes in blood oxygenation. By contrast, it would be expected that the veins receiving blood from active tissue would absorb less light or become brighter under illumination 660 nm light. This follows from the facts that there would be an increase in HbO2 in the venous network, and HbO2 absorbs less than Hb at this wavelength ( Fig. 41.1A ). These hypotheses have recently been tested and validated in the monkey cortex2 ( Fig. 41.1B and 41.1C ). IOS imaging was performed in those studies at sufficiently high magnification to reveal the optical changes occurring within the microscopic pial arterioles and surrounding venous network during electrical stimulation of the cortical surface. It can be seen that the optical changes at 535 nm are restricted to the dilating pial arterioles that absorb more light after cortical activation. At 660 nm, the optical changes are restricted to the nearby veins that become brighter at the same time ( Fig. 41.1C ). These experiments suggest optical changes at 535 nm are restricted to the dilating pial arterioles nearest to the neurons that fire action potential; hence, IOS imaging at this wavelength would be expected to generate maps that reliably localize cortical areas of increased activity. Optical changes at 660 nm are localized to the venous network, which receives blood from active cortical areas that is more highly oxygenated during increased neuronal activity. Because the more oxygenated blood may flow into vessels that may be located at various distances from the site of activation, it would be expected that IOS imaging at 660 nm would be less reliable for localizing active cortical areas. In summary, these recent studies suggest that IOS-imaging at 535 nm, which reflects activity-evoked changes in blood volume, may be an accurate and reliable method for intraoperative cortical mapping.


Intraoperative IOS imaging studies on human subjects further support the notion that IOS imaging of blood volume changes at 535 nm can be used to reliably map functional and epileptic activity.1 We have recently performed a more extensive series of careful studies on the use of 535 nm IOS imaging for localizing cortical activity. One experimental method for investigating that ability of IOS imaging for mapping epileptic activity involves electrical stimulation of the cortex at various currents that are either below or at the threshold for eliciting after-discharge activity, which is electrophysiologically similar to ictal discharges ( Fig. 41.2 ). Through stimulation of the cortex for 4-second durations with an Ojemann Stimulator (Integra Lifesciences Corporation, New Jersey, USA; 60 Hz, biphasic), it is typical to find stimulation currents that are just sufficient to reliably and repeatedly elicit after-discharges. Stimulation at a current that is just 1 mA below this after-discharge threshold will fail to elicit any after-discharges. Fig. 41.2A shows data from one such experiment in which 6 mA was found to be the after-discharge threshold. In these experiments, it is found that after-discharge activity generates optical changes at 535 nm that are of significantly greater magnitude and of longer duration than optical changes elicited by neuronal activity below the after-discharge threshold. Further, the optical changes are observed to spread into cortical areas that are more distant from the stimulating electrodes during the after-discharge activity. These observations suggest that epileptic activity may have a distinctly different optical signature from nonepileptic activity. Intraoperative IOS imaging in combination with electrical stimulation may thus provide a reliable means to map epileptogenic cortical tissue.
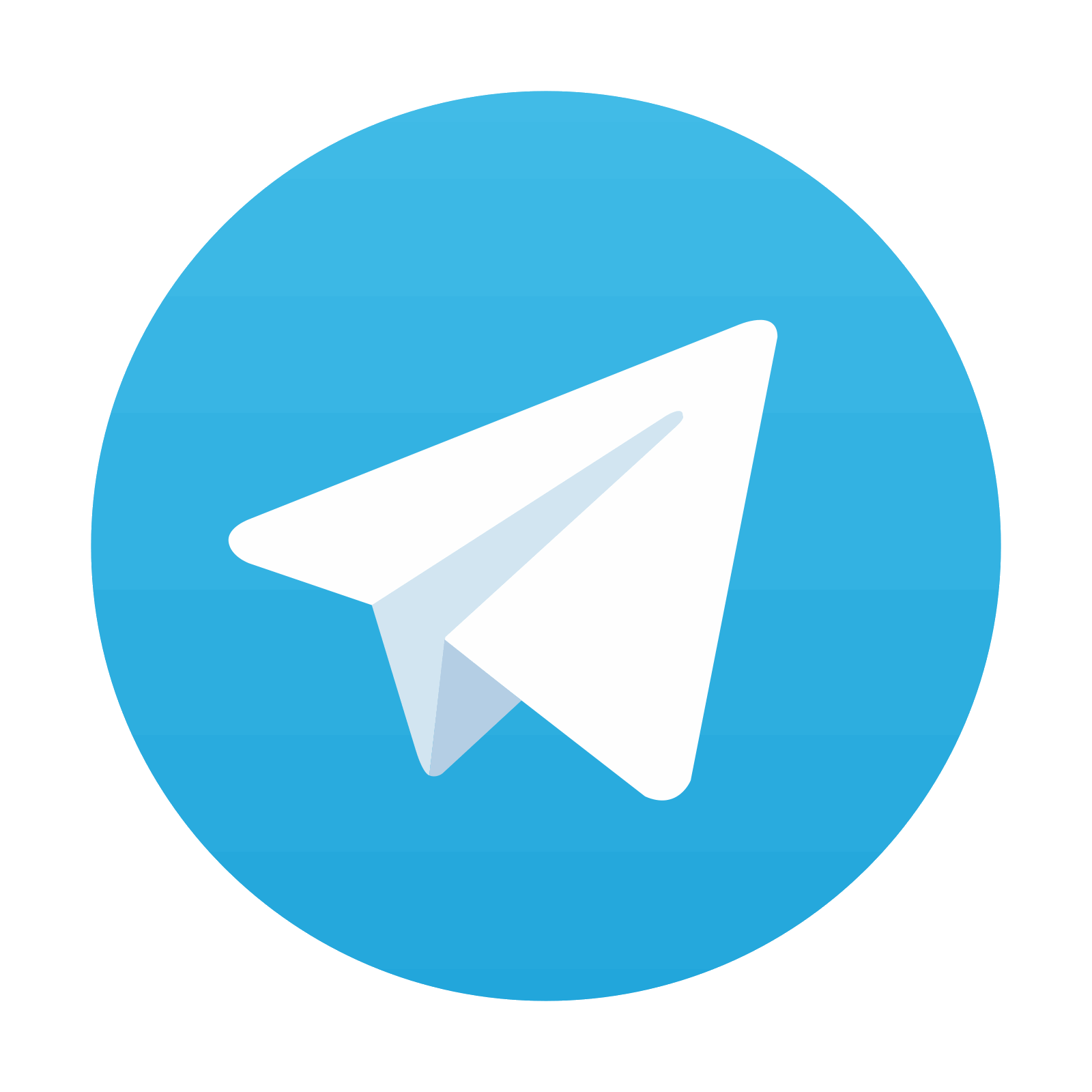
Stay updated, free articles. Join our Telegram channel
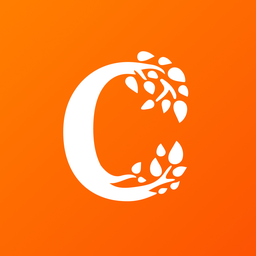
Full access? Get Clinical Tree
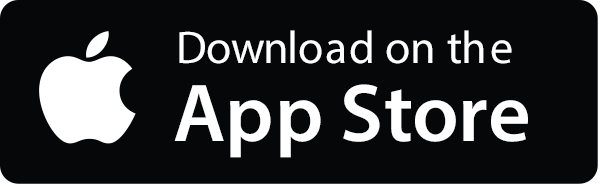
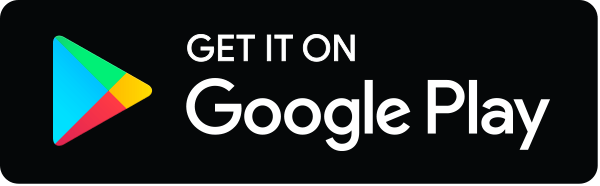