Fig. 26.1
(a) The structure of adenosine 5′-triphosphate (ATP) and ATP derivatives. (b) Schematic representation of purine metabolism from ATP to uric acid. ATP is degraded to hypoxanthine, which can be salvaged by hypoxanthine–guanine–phosphoribosyl–transferase (HGPRT) or further metabolized to xanthine and uric acid by xanthine oxidase. Enzymes are in italics. Guanine-based purines (GTP to guanosine, guanine and xanthine) are not illustrated. Allopurinol inhibits the enzyme xanthine oxidase, possibly favoring purine salvage. Purinergic system and sources of extracelular adenosine. ATP stored in presynaptic vesicles is released after depolarization, acting on P2 receptors and being dephosphorylated by ecto-NTPDases and ecto-5′nucleotidase (ecto-5′NT) into adenosine (ADO). ADO acts mainly on A1R and A2AR and can be converted into inosine extracellularly by ecto-adenosine deaminase (ecto-ADA) or uptaken by nucleoside transporters. Inside the cell, ADO preferentially forms AMP by adenosine kinase (AK) but also may be deaminated by ADA (not shown). The sources of extracellular adenosine are ATP, adenosine released as such by nucleoside transporters (in situation of energetic imbalance, such as brain damage), and cAMP, which is converted into AMP by an ecto- phosphodiesterase and later into ADO
Adenosine consists of a purine base (adenine) attached to the 1′ carbon atom of ribose (Fig. 26.1a). As mentioned above, this ribonucleoside is mostly produced by ATP catabolism, both at the intra- and extracellular level (Fig. 26.1b), although to a lesser extent it can also be generated by S-adenosyl-l-homocysteine (SAH) metabolism (Fig. 26.1b). Once formed, adenosine can be released either through Na+-dependent transporters or intracellularly phosphorylated to form AMP by the action of adenosine kinase (Fig. 26.1b). In addition, adenosine can react with l-homocysteine to form SAH (Fig. 26.1b). Finally, adenosine can be deaminated to 6-1B. Interestingly, adenosine has been historically considered a retaliatory metabolite [8] that increases oxygen supply and decreases oxygen consumption, thus modulating a large array of physiological processes. In such a way, adenosine impinges on respiratory function [9], neural activity [10], platelet aggregation [11], neutrophil function [12], lymphocyte differentiation [13], and vascular tone [14]. Additionally, adenosine is able to provoke both coronary arteries dilatation and kidney blood vessels contraction, thus reducing renal filtration [15]. Adenosine also exerts a negative chronotropic and dromotropic effect on the heart [16] and mediates the inhibition of neurotransmitters release [17] and lipolysis [18]. Accordingly, it has been theorized that this purine nucleoside is a mediator of metabolic distress, thus having considerable impact on homeostatic cellular functioning.
On the other hand, adenosine has been shown to play a key regulatory role in the CNS, thus acting as a presynaptic, postsynaptic, and/or non-synaptic neuromodulator [19]. Extracellular adenosine levels in the brain range high nM concentration at basal conditions and are related to the intracellular concentration of adenosine and nucleotides such as ATP, AMP and cAMP [20]. Indeed, the intracellular adenosine concentration is related to the rate of breakdown and synthesis of ATP [20]. Thus, adenosine is released as a neuromodulator [21] by the effector cells in response to an increased metabolic demand [22]. Interestingly, it has been proposed that the main source of extracellular adenosine in the striatum comes from intracellular cAMP [23], which is metabolized to AMP by means of phosphodiesterases and then to adenosine by the ecto-nucleotidases (Fig. 26.1b). Overall, because cAMP can only be generated by the action of the enzyme adenylyl cyclase, striatal extracellular adenosine would mostly reflect an increased activation of receptors positively linked to adenylyl cyclase.
Adenosine Receptors in the CNS
In 1972, it was shown that electrical stimulation of brain slices promoted adenosine release [24]. Interestingly, this stimulated release of endogenous adenosine concomitantly produced cAMP intracellular accumulation, a fact that was blocked by methylxanthine (i.e., caffeine and theophylline) incubation [25]. This phenomenon was also observed in other tissues such as the heart [26]. Collectively, these observations constituted the first piece of work suggesting that extracellular adenosine exerted its effects via specific plasma membrane receptors. It was later demonstrated that the adenosine-mediated antilipolytic effect on fat cells occurred with a concomitant reduction in cAMP [27]. This dual effect of adenosine on cAMP formation was further substantiated when it was demonstrated that adenosine could either inhibit or stimulate adenylyl cyclase. Overall, these observations concluded with the first subclassification of adenosine receptors into Ri and Ra [28], or alternatively, A1 and A2 adenosine receptors [29].
It is currently well established that adenosine mediates its actions by activating specific G protein-coupled receptors (GPCRs), for which four subtypes (A1R, A2AR, A2BR and A3R) have been identified to date. These receptors have a distinguishable pharmacological profile, tissue distribution, and effector coupling [30], and its functioning has been largely studied in the CNS (Table 26.1). Adenosine receptors belong to the rhodopsin family, or class A of GPCRs [52], thus sharing some common molecular features. For instance, within their sequence, all adenosine receptors contain the widely conserved NPxxY(x)5,6 F and the DRY motifs [53, 54]. Thus, adenosine-mediated conformational rearrangement of adenosine receptors determines the binding and activation of specific G proteins (Table 26.1) which are responsible for activation of different intracellular signaling pathways associated to adenosine function (Table 26.1).
Table 26.1
Adenosine receptors
Receptor | Adenosine affinity | G protein | Transduction mechanismsa | Physiological actions |
---|---|---|---|---|
A1 | ~70 nM | Gi/o b Gq/11 Gs | •Inhibits: ACb •Activates: PLC, AC | |
A2A | ~150 nM | Gs b Golf G15,16 § | •Activates: ACb, PLC •Inhibits: Ca2+ channels | |
A2B | ~5,000 nM | Gs b Gq/11 | •Activates: ACb, PLC | |
A3 | ~6,500 nM | Gi/o b | •Inhibits: ACb •Activates: PLC |
A1Rs and A2ARs are primarily responsible for the central effects of adenosine (Table 26.1) [55]. The most abundant and homogeneously distributed adenosine receptor within the brain is the A1R, which is functionally coupled to members of the pertussis toxin-sensitive G proteins (Gi1, Gi2, Gi3 and Go) and whose activation regulates several intracellular effector molecules (i.e., adenylyl cyclase, Ca2+ channels, K+ channels, and phospholipase C; Table 26.1) [56]. Conversely, A2AR is expressed at high levels only in some specific brain regions (e.g., striatum, olfactory tubercle, and nucleus accumbens) [23, 57]. A2ARs are mainly coupled to Gs/Golf proteins [58], thus activating adenylyl cyclase and increasing intracellular cAMP levels (Table 26.1). Interestingly, A2AR may also signal through a G-protein independent pathway eventually associated to mitogen-activated protein kinase signaling cascade activation [59]. Next, the A2BR is positively coupled to adenylyl cyclase and phospholipase C (PLC) through Gs and Gq proteins, respectively (Table 26.1) [2]. A2BR is thought to be fairly ubiquitous in the brain, and the association of this receptor to specific physiological or behavioral responses remains quite scarce because the A2BR-specific pharmacological tools are limited [60]. Finally, A3R has been shown to be coupled to Gi/o proteins, thus inhibiting adenylyl cyclase and also stimulating PLC (Table 26.1) [2].
The Adenosine Hypothesis of Schizophrenia
Schizophrenia comprises a heterogeneous group of syndromes of unknown etiology. It is a serious mental disorder affecting up to 1 % of the population worldwide that usually arises during late adolescence and early adulthood (i.e., median age onset is about 23 years in men and 28 years in women). The definition of schizophrenia has evolved throughout the five editions of the Diagnostic and Statistical Manual of Mental Disorders (DSM) published by the American Psychiatric Association. For instance, in the DSM-IV version published in 1994, schizophrenia was defined as a mental disorder involving a range of cognitive and emotional dysfunctions that include perception, inferential thinking, language and communication, behavioral monitoring, affect, fluency and productivity of thought and speech, hedonic capacity, volition and drive, and attention. The diagnosis involved the recognition of a constellation of signs and symptoms associated with impaired occupational or social functioning: no one symptom was pathognomonic of the disorder. Additionally, in the fourth version the pathology had high diagnostic stability, with 80–90 % of individuals receiving an initial diagnosis of schizophrenia and retaining that diagnosis at 1–10 years [61, 62]. However, in the current DSM-5 version, five characteristic symptoms for the diagnosis of schizophrenia are established with the requirement that at least two of the symptoms be present for a month [63]. Three changes with regard to the previous version have been made and include the elimination of the special treatment of bizarre delusions and Schneiderian “first-rank” hallucinations, clarification of the definition of negative symptoms, and the addition of a requirement that at least one of the minimum two requisite characteristic symptoms must be delusions, hallucinations, or disorganized speech [63]. Of note, the current classification seeks to incorporate the new information about the nature of the disorder accumulated over the past two decades. Thus, the disease is now considered to be characterized by positive, negative, and cognitive symptoms. Positive symptoms reflect the appearance of some phenomena that were not present in the past, and include hallucinations and delusions. On the other hand, negative symptoms, such as anhedonia or apathy, reflect the loss of capacities or characteristics previously gained. Finally, the cognitive symptoms include alterations in attention, working memory, executive functions, and social cognition.
Several theories about the neurotransmission systems affected in schizophrenia have been presented. Thus, nearly all known neurotransmission systems (i.e., dopaminergic, glutamatergic, serotoninergic, GABAergic, and cholinergic) have been in one way or another involved in schizophrenia, although none of those theories fully explains the fundamental pathological process(es) associated with the disease. Interestingly, although they were initially proposed as separate hypothesis (i.e., the “glutamatergic” and the “dopaminergic” hypothesis) [64, 65], one of the most currently supported theories is based on a combined hyperdopaminergic-hypoglutamatergic phenomenon [66]. Indeed, current pharmacotherapy for schizophrenia is based on a “dopamine” and “glutamate” hypothesis, which is centered in a striatal dopamine D2 receptor (D2R) hyperfunction, a deficient stimulation of prefrontal cortex (PFC) dopamine D1 receptors (D1Rs) and a N-Methyl-d-aspartate (NMDA) receptor hypofunction in the PFC [67]. However, because negative symptoms, cognitive dysfunction, and decrements in psychosocial and vocational functioning often are still persistent on available pharmacotherapy, the development of a next generation of pharmacologic agents tackling these resilient symptoms is needed [68]. Overall, additional research in non-dopaminergic and non-glutamatergic alternatives is necessary to improve the caveats in schizophrenia treatment.
As mentioned above, adenosine plays an important role in the CNS both as a homeostatic neuronal bioenergetic mediator and as a neuromodulator agent. Indeed, an adenosine-mediated modulation of dopaminergic and glutamatergic neurotransmission has been described [69–71]. Thus, adenosine agonists and antagonists produce behavioral effects similar to those of dopamine antagonists and dopamine agonists, respectively [72]. Additionally, adenosine tone can also modulate glutamatergic neurotransmission [73, 74]. Hence, adenosine may play a unique role integrating glutamatergic and dopaminergic neurotransmission systems. Accordingly, a purinergic hypothesis of schizophrenia was proposed by Lara and co-workers longer than a decade ago [75]. The authors theorized that a dysfunction in the purinergic system (i.e., reduced adenosinergic activity) would account for the imbalance observed between dopaminergic and glutamatergic neurotransmission, a phenomenon that would explain the schizophrenia phenotype [76]. Indeed, several experimental sources of evidence supported the adenosine hypothesis of schizophrenia, which will be highlighted in this chapter.
Experimental Evidence: Preclinical Models of Schizophrenia
In general, a considerable lack of knowledge still exists concerning psychiatric illnesses. Of note, this incomprehension is especially remarkable in the case of a highly intricate pathology as is schizophrenia. For that reason, animal models eventually mimicking some of the schizophrenia-associated symptoms may not precisely mirror what exactly occurs in a schizophrenic human patient. However, preclinical models can be valuable experimental tools to shed light on the mechanisms behind the etiopathology of schizophrenia. As mentioned above, the adenosinergic hypothesis of schizophrenia was proposed to interconnect both schizophrenia-associated dopaminergic hyperfunction and glutamatergic hypofunction. Indeed, some experimental sources of evidence obtained from experimental animal models supported the adenosine contribution to schizophrenia through the modulation of both dopaminergic and glutamatergic neurotransmission [77]. Hence, we will review the animal models supporting the glutamatergic hypofunction theory (e.g., phencyclidine model) and the hyperdopamergic hypothesis (e.g., amphetamine model) and its relationship with adenosinergic neurotransmission.
The formulation of the hypoglutamatergic-NMDA receptor hypothesis appeared in the late 1950s, when it was observed that phencyclidine (PCP) provoked a psychotic-like condition similar to that observed in schizophrenic patients [78]. However, no one suspected that NMDA receptors were behind this phenomenon until the 1980s when Lodge and colleagues [79] demonstrated that NMDA receptor blockade was in fact the primary mechanism of PCP-mediated psychotic actions. Indeed, blockade of NMDA receptors promoted both glutamate and dopamine release in the PFC [80], thus disrupting glutamatergic and dopaminergic neurotransmission in this brain region. It was then believed that the neurotransmitter imbalance could be well correlated with the cognitive and behavioral perturbations observed in schizophrenia [80–82]. Interestingly, the administration of NMDA receptor antagonists either in the late fetal or in the postnatal period of rats was shown to increase the neuronal death by apoptosis [83], a phenomenon that would be linked to adult schizophrenia-like behavior. On the other hand, administration of the same type of compounds in the adult animal increased the neuronal damage by necrosis with the subsequent gliosis [84], also associated with psychotic-like behavior. In conjunction, these experimental observations substantiate a neurodevelopmental link between NMDA receptor antagonists and schizophrenia. Thus, the hypoglutamatergic-NMDA receptor theory suggests the existence of disturbances in the pre- and perinatal brain development that could provoke clinical manifestations in early adult life [85]. Nevertheless, despite the experimental evidence and some clinical observations, the precise mechanism involving NMDA receptors in schizophrenia is still unknown.
The hypofunction of NMDA receptors in adults, root of the glutamatergic hypothesis of schizophrenia [86], has been historically sustained by pharmacological animal models using NMDA receptor antagonists (i.e., PCP, ketamine, and dizocilpine). PCP is a dissociative drug first synthesized in 1926 as a surgical anesthetic. Despite its efficacy, the use of the drug was not prolonged because of its concomitant adverse effects (e.g., hallucinations, delusions, and agitation). Thus, because PCP mimics some schizophrenia symptoms in humans it has been extensively used in animals as a model for the illness. Indeed, acute administration of PCP produced hyperlocomotion [87], social withdrawal [88], and failures both in cognition [89] and in sensorimotor gating [90] in rodents. On the other hand, chronic PCP treatment also promoted hyperlocomotion as indicative of positive symptoms. Furthermore, chronic treatment induced deficits in social behavior and reduced mobility in the forced swimming test, which corresponds to negative symptoms. As for the cognitive symptoms, PCP-treated animals displayed sensorimotor gating deficits and cognitive dysfunctions when subjected to learning and memory tests [91]. Interestingly, these PCP-mediated schizophrenic-like symptoms were maintained in humans for several weeks following chronic treatment [92, 93]. Thus, the PCP-induced model of schizophrenia seems to partially resemble the pathology, although some criticisms exist regarding this PCP-based animal model. For instance, sensorimotor deficit in the prepulse inhibition test does not last after PCP withdrawal in animals, which differs from the human disease; some discrepancies have been reported regarding negative symptoms between clinical features and PCP-treated animals [91].
Interestingly, adenosine receptors have been shown to be able to modulate psychostimulant effects in PCP-treated animals. Hence, both A1R and A2AR agonists (i.e., CPA and CGS21680, respectively) were able to counteract PCP-mediated hyperlocomotor activity [94, 95], while A2AR blockade, but not A1R, prompted exhacerbation of the motor-estimulant effects of the NMDA antagonist [96]. PCP-induced psychomotor activities were enhanced in a KO mouse specifically lacking the striatal neuronal A2AR [97]. However, an opposite effect was observed in a knockout mouse lacking the forebrain A2AR with the A2AR deleted in the neurons of the striatum and the cerebral cortex and hippocampus. Accordingly, a critical role for A2ARs in extrastriatal neurons was described in providing a major excitatory effect on psychomotor activity [97]. Overall, these results indicate that A2ARs in striatal and extrastriatal neurons exert an opposing modulation of psychostimulant (i.e., PCP-mediated) effects.
Similarly to the hypoglutamatergic-NMDA receptor hypothesis, the dopaminergic hypothesis of schizophrenia had several important conceptual changes throughout its history. It was initially based on a generalized hyperdopaminergic brain function, but quickly evolved into a combined subcortical hyperdopaminergic-prefrontal hypodopaminergic dysfunction. Nevertheless, Howes proposed an updated third version based on multiple changes of different neurotransmitters and neural systems, which with other biological or environment influences, would underlie the cognitive dysfunction and negative symptoms of schizophrenia. In Howes’ words, rather than being a hypothesis of schizophrenia this new view is more accurately a “dopamine hypothesis of psychosis-in-schizophrenia”. This hypothesis explains several environmental and genetics risks for schizophrenia and proposes that these interact to funnel through one final common pathway of presynaptic striatal hyperdopaminergia [98].
The hyperdopaminergic status of schizophrenia has been largely studied through means of pharmacological animal models. Thus, the administration of drugs (i.e., amphetamine) that increase the brain dopamine content is a classical experimental approach to study schizophrenia-associated positive symptoms. Amphetamine, first discovered in 1887 [99], is currently used as an attention deficit (i.e., attention deficit hyperactivity disorder) and narcolepsy treatment [100]. It is a drug that acts as a strong CNS stimulant by increasing dopamine concentration in the synaptic cleft, thus raising the response in the postsynaptic neuron. Apart from the well-known positive effects, its administration could also provoke long-term cognitive impairments [101, 102]. Overall, while several investigations have demonstrated that amphetamine treatment could induce some behavioral, molecular, cellular and neurochemical changes which were behind the striatal dopaminergic system [103–106], the studies reporting amphetamine-mediated negative symptoms are scarce.
Dopamine receptors on striatonigral and striatopallidal neurons (D1R and D2R, respectively) play a pivotal role in the control of motor responses [107], thus the efficacy of many antipsychotic drugs correlates well with their ability to block D2Rs [108]. Because A2ARs antagonistically interact with D2Rs [109, 110], adenosine is expected to exert a regulatory influence on psychomotor behavior, and indeed a role for A2AR regulating amphetamine-induced psychomotor behavior has been described [111]. Thus, A2AR activation restored responsiveness to amphetamine in adenosine-deficient mice [111]. The abovementioned preclinical data have supported the involvement of adenosine in schizophrenia and the potential use of adenosine receptors as drug targets for this disease.
Clinical Evidence Supporting the Adenosine Hypothesis
Several lines of investigation support that the adenosinergic system can be altered in schizophrenia. The first striking piece of information pointing to this consists of the finding that A2AR expression was shown to be increased in necropsies from schizophrenic individuals [112]. Because the adenosinergic tone was shown to be reduced in schizophrenia, A2AR up-regulation could correspond to an adaptive physiological condition that in turn would be associated to a concomitant hyperdopaminergic state [76]. Interestingly, the genetic linkage of adenosine receptors to schizophrenia has been somehow evaluated. While an A2AR genetic variant (i.e., 1976 T > C) was not shown to confer susceptibility to schizophrenia [113], the involvement of some A1R gene polymorphisms in the pathophysiological mechanisms of schizophrenia was suggested [114]. In addition, the most frequent functional polymorphism of adenosine deaminase (22G → A, ADA1*2), which is characterized by a reduced enzymatic activity and thus higher adenosine levels, is less frequent among schizophrenic patients [115]. Collectively, these results support the hypothesis of lower adenosinergic activity in schizophrenia.
Based on prior data, it seems feasible to think that the use of pro-adenosinergic drugs could be beneficial for the treatment of the pathology. However, this pharmacological proposal is still vague although some data exist regarding this hypothesis. Indeed, raising the endogenous pool of purines with allopurinol has been shown to produce promising results as add-on therapies for schizophrenia [116, 117]. Allopurinol is a well-known hypouricemic drug that inhibits xanthine oxidase (Fig. 26.1b) and was used as an add-on drug in the treatment of poorly responsive schizophrenic patients [116]. In a short controlled trial (i.e., 23 patients treated with haloperidol 15 mg/day plus allopurinol 300 mg/day and 23 patients with haloperidol 15 mg/day plus placebo), it was observed that the combination of haloperidol and allopurinol showed a significant superiority over haloperidol alone in the treatment of positive symptoms, general psychopathology symptoms, as well as the Positive and Negative Syndrome Scale (PANSS) total scores [116]. In a similar study, a double-blind, placebo-controlled, crossover clinical trial of add-on allopurinol (300 mg/day) for poorly responsive schizophrenia or schizoaffective disorder (DSM-IV criteria), was conducted on 22 patients [117]. Allopurinol was an effective and well-tolerated adjuvant treatment, particularly for refractory positive symptoms [117]. Allopurinol also showed effectiveness as an adjunctive medication in schizophrenia outpatients (N = 59) with persistent symptoms despite adequate pharmacotherapy [118]. In a recent case report, allopurinol prompted a rapid decrement of psychotic symptoms in a patient with schizophrenia [119]. Thus, within 2 weeks of allopurinol adjuvant therapy, the patient showed significant improvement with respect to positive and negative symptoms of schizophrenia (PANSS scores went from 88 to 41 within 2 weeks) [119]. Collectively, these clinical studies suggest that allopurinol might be an effective adjuvant drug in the management of patients with chronic schizophrenia who are poorly responsive to current treatments. However, larger, randomized clinical trials are needed before a broad clinical application of allopurinol is recommended as a routinely used adjuvant therapy to antipsychotics [120]. Another piece of evidence supporting the link between the adenosinergic system and schizophrenia consists of the fact that the adenosine transport inhibitor dipyridamole was found to be beneficial in patients with schizophrenia [121]. Thus, raising extracellular adenosine levels with dipyridamole not only improved haloperidol-mediated amelioration of positive and general psychopathology symptoms as well as PANSS total scores [121], but it also showed effectiveness when combined with lithium in the treatment of acute bipolar mania [122].
Although some clinical controversy has been established around allopurinol [123], the adenosine modulator adjuvant therapy was shown to be beneficial in overall psychopathology (especially positive symptoms) in schizophrenia and in treating mania episodes of bipolar disorder when compared with placebo [124]. However, larger and superior clinical trials are needed to undeniably sustain the use of these drugs in schizophrenia. Overall, all the abovementioned clinical data support the adenosine hypothesis of schizophrenia and highlight the potential pharmacological interest of combining antipsychotic drugs with a purinergic-based compound (i.e., allopurinol) to tackle resilient schizophrenia symptoms.
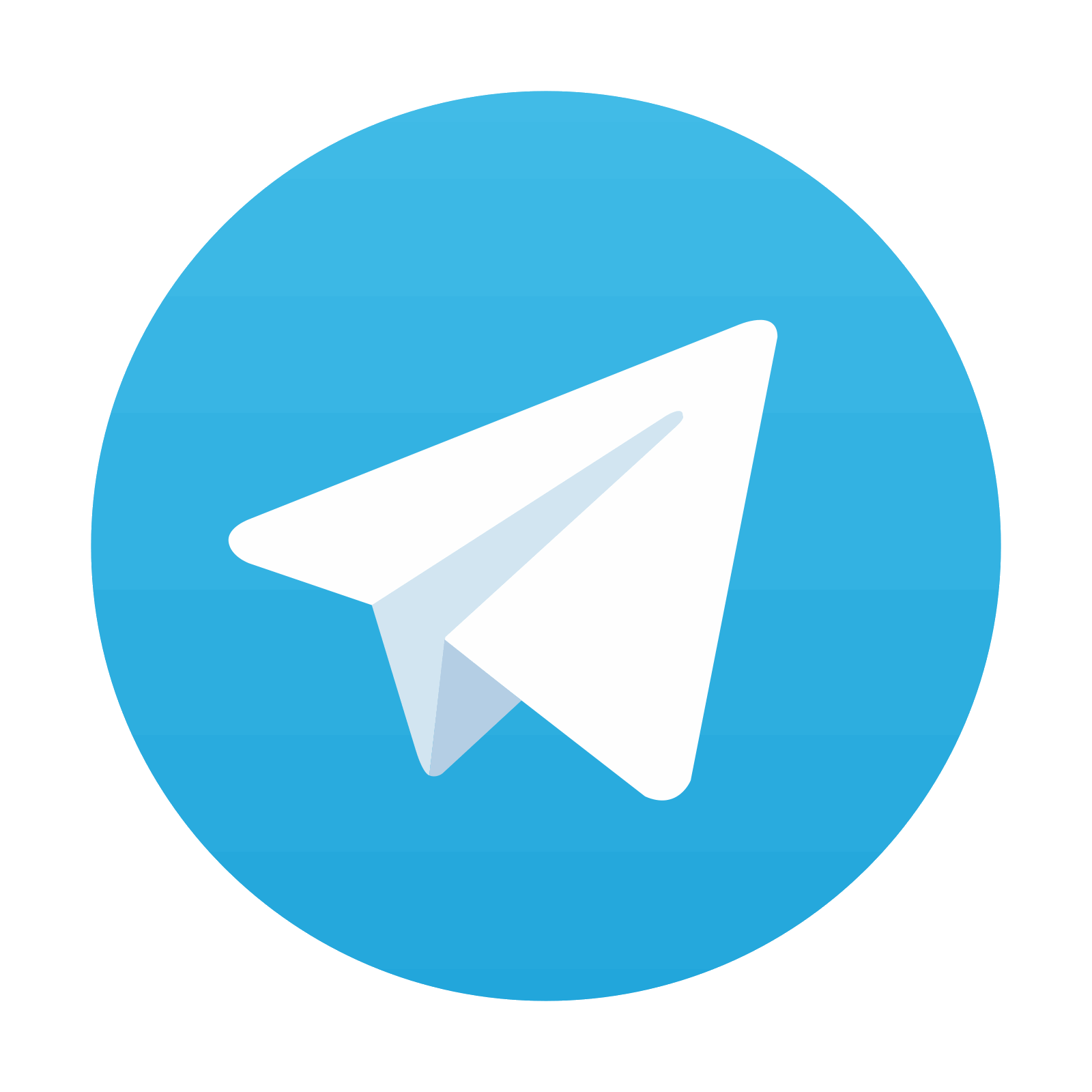
Stay updated, free articles. Join our Telegram channel
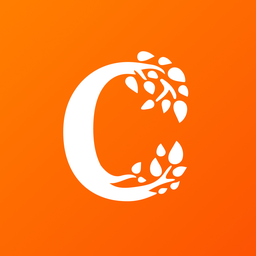
Full access? Get Clinical Tree
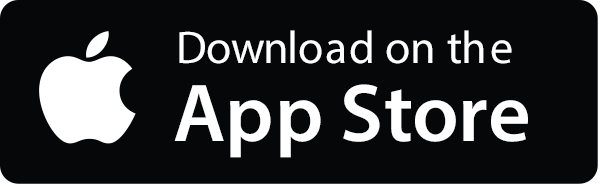
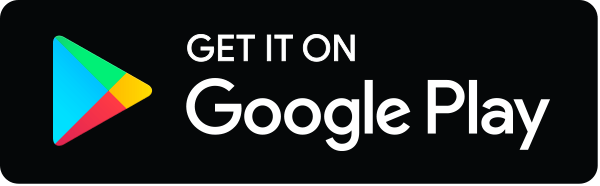