1 Applied Neurophysiology 1.1 Systems Control: Systems and Structures Concerned with Movement and Sensorimotor Integration 1.3 Consequences of and Reorganization after CNS Lesions Knowledge of the functions of the central nervous system (CNS) has historically been drawn from experimental studies on animals. In recent years, developments in movement science have led to studies being undertaken mostly on normal, healthy people. More recently, advances in noninvasive neuroimaging techniques have made it possible not only to study local changes in the brain function of people with CNS lesions but also to follow changes in the CNS over time. Techniques such as functional magnetic resonance imaging (fMRI), positron emission tomography (PET), transcranial magnetic stimulation (TMS), electroencephalography (EEG), and magnet encephalography (MEG) show changes in the structure of the brain and how these correlate with changes in the patient’s physical functioning post-lesion (Academy of Medical Sciences 2004, Ward and Cohen 2004). Knowledge about neurophysiology, normal movement, and deviations from normal movement forms the basis for clinical reasoning. This chapter therefore aims to discuss movement and the post-lesion alterations in movement and covers: • Systems control: Systems and structures concerned with movement and sensorimotor integration – The neuromuscular system: The muscular system, plasticity, communication with the spinal cord – The somatosensory system, vision, and balance: The connection between somato-sensory system, vision, and movement in relation to stereognostic sense and balance – Systems within the spinal cord and brain: Systems important for movement and analysis of movement in a clinical situation • Plasticity: How the brain is structurally and functionally modified by the information it receives • Lesions and reorganization: Consequences of damage to the CNS The chapter includes a simplified presentation of the aspects of CNS function that are particularly relevant in the production and development of sensorimotor function. The reader is advised to review other relevant publications for revision and more in-depth information (e. g., Brodal’s The Central Nervous System and Kandel et al.’s Principles of Neural Science). The integrated model of human movement, in which all systems—sensory, motor, perceptual, and cognitive—have important roles in making movement efficient and in the context of the individual will be discussed. No system works in isolation; all systems—network with other systems, they receive, integrate, and pass on information, and they affect and are affected by other systems. “Movement is the output of a hybrid functional system interlinked to its environment in which sensory, cognitive and motor processes interact” (Mulder et al. 1996). Motor behavior is the result of integration between the individual, the task, and the environment in which action is being performed. Different systems have different roles in different contexts to make behavior appropriate to the moment. Localizing CNS function is a complex task and has undergone huge advancements from the science of phrenology, in which “bumps” on a person’s skull were thought to represent a specially developed brain area, to imaging techniques that permit us to see the human brain in action. Different regions of the brain are specialized for different functions and yet the organization of the CNS is known as parallel distributed processing. Many sensory, motor, and cognitive functions are served by more than one pathway. To some degree, this helps regions or pathways to partially compensate for each other if damage occurs (Kandel et al. 2000). The structure and function of the muscular and nervous systems have to be discussed together. CNS and motor activity influence each other. Movement is the end result of the action of skeletal muscles as a result of CNS processing. Processing within the CNS is a result of information sent to the system about the desire or requirement to act, based on a need to interact with the environment. The individual, the functional task, and the environment in which movement is being performed require appropriate processing and function within the CNS and muscular systems. The muscular system and the CNS exchange information and demands continuously. The muscular system is specialized in its structure and function to meet the needs of a variety of movements in different settings to perform a multitude of tasks. The neuromuscular system has an adaptive capacity. Changes in the information sent by the CNS to the muscular system may alter the structure and function of the musculature, and vice versa (altered use of the muscles due to CNS lesions may result in changes in the structure and function of the CNS). Thus, on the basis of the interdependence between these systems, this chapter is called “The Neuromuscular System.” Skeletal muscle contains contractile and noncontractile elements and specialized sense organs or receptors. The contractile elements are the extra-fusal muscle fibers and ends of the muscle spindles. The noncontractile elements are the connective tissue and sense organs (the Golgi tendon organ and muscle spindles). Muscle tone is related to the state of muscle fibers, the activity of the sense organs, viscosity, and the state of the fibrous tissue. These are divided into three main groups and several subgroups. The three main groups are: • Type 1, also called ST (slow twitch; Brodal 2001) or SO (slow oxidative; Kidd et al. 1992, Rothwell 1994). This fiber type is often described as red due to its high content of myoglobin. Type 1 fibers have a high level of endurance, are precise in their action, and produce a moderate amount of power. The action of these fibers is often referred to as tonicity due to the ability of the fibers to maintain dynamic contraction over time; they are mostly found in the areas of the body where maintenance of activity against gravity is the main function. They have a stabilizing function through their precise grading of activity. Tonic activity is dynamic, and the word tonicity refers to something that is “characterized by tension or contraction, esp. muscular tension” (Taber’s Cyclopedic Medical Dictionary 1997). The motor units containing type 1 fibers are characterized as S (slow to fatigue; Rothwell 1994). The soleus muscle is continuously active during standing and walking. Therefore it needs to have high endurance and consists of tonic/type 1 motor units. The soleus muscle is characterized as a postural muscle. The small muscles of the hand, the interossei, and the lumbricals also mostly have type 1 fibers (Rothwell 1994). The small muscles of the hand stabilize the palm and the metacarpophalangeal joints and provide the hand with a postural background for individual finger movement and precise fine motor control. The small muscles of the foot have a stabilizing function for maintenance of equilibrium of the body. The small muscles of the back are important for postural control of the trunk and therefore core stability. • Type 2 fibers are also called FT (fast twitch) because they have a faster contraction speed than type 1 fibers. These muscle fiber types are described as white and have a low oxidative capacity with little endurance but increased tempo and force production. They are phasic by nature, and their main function is production of movement. The motor units are classified as FF (fast, fatigable). Type 2 fibers are further subdivided into: – Type 2A or FOG (fast oxidative glycolytic; Rothwell 1994). The motor units are classified as FR (fatigue resistant) because they have more endurance Example The gastrocnemius muscle has an important role in force production during locomotion, running, jumping, moving on uneven ground, and climbing steps, which require both endurance and force. The muscle therefore has a larger proportion of FOG muscle fibers. – Type 2B are the truly white fibers (Brodal 2001). The motor units are classified as FG (fast glycolytic; Rothwell 1994) and have low endurance and produce a lot of force Example The tibialis anterior muscle works intermittently during locomotion and in standing. Its use therefore is mainly aimed at phasic activity and low demands of endurance. Muscle fibers are able to alter their fiber type to some degree in relation to use (Brodal 1998). At birth most muscles are composed of slow (type 1) muscles and only as the body matures does the final proportion of slow and fast muscles emerge (Langton 1998). Athletes have different distributions of muscle fiber types depending on their preferred sport. Long-distance runners, cyclists, and cross-country skiers have a larger percentage of type 1 red fibers, whereas weight-lifters, short-distance runners—sports that require rapid production of force—have a larger percentage of white type 2 fibers. Partly, this is probably due to the individual genetic profiles but muscle plasticity forms a major basis for the physiologic adaptation to our external environment. Examples of muscle plasticity are adapting to exercise, effects of a microgravity environment, aging, and different pathophysiologic conditions. Muscle plasticity can be both beneficial and maladaptive. Muscle cells display a tremendous ability to adapt to new levels of gene expression in response to a wide range of environmental demands and clinical conditions (Sieck 2001). Gene expression is the process by which a gene’s information is converted into the structures and functions of a cell (Wikipedia 2006). Alteration of muscle fiber types is a result of changed gene expression. This may be termed use-dependent plastic adaptation. Trials using electrostimulation have demonstrated that muscle fibers may change if the information to the muscle fibers and functional demand are altered (Kidd 1986, Langton 1998). Muscles are able to alter their fiber type to some degree in relation to use. Contraction of skeletal muscle is caused by gradual overlap of actin and myosin filaments within the muscle cell or fiber. A muscle fiber is composed of many areas of overlapping myosin and actin called sarcomeres. The numbers of sarcomeres determine the length of a muscle fiber: the more sarcomeres, the longer the muscle. Normally, in the human body the number of sarcomeres, and therefore the length of the muscle, is optimal for the function of the muscle. The force of contraction is therefore at its best within the range of movement where it is most needed. The sarcomeres are able to produce very little force if the fiber is overstretched or kept in a much-shortened position. The length of the muscle fibers is affected by the way the muscle is being used. If a muscle is kept in a much-shortened position over time, this may lead to an anatomic shortening due to loss of sarcomeres. Sahr-mann (1992) states that a shortened muscle is more easily recruited than its antagonists which are in a lengthened position, and that as a result of this, the shortened muscle is stronger. She calls this biased recruitment. If a muscle is kept stretched over time, it may start “growing,” that is, the number of sarcomeres may increase. This may cause the muscle to be too long to be able to produce the appropriate amount of force needed for an activity. Sahr-mann (1992) calls this stretch weakness. The length of a muscle is important for movement and function. A motor unit comprises of an anterior horn cell (α-motor) with its axon and the muscle fibers it innervates (Fig. 1.1). All muscle fibers in a motor unit are of the same type, and within any muscle there are many other motor units with different fiber type representation. All contact between the CNS and the muscle is through the peripheral nervous system. Brodal (2001) states that motor units vary in size depending on the size and function of the muscle; there may be fewer than 10 muscle fibers within a unit, such as the external eye muscles, or more than 1000, as in the large muscles of the back. The number and size of the motor units vary. Smaller units are found in muscles that perform precise movements with moderate strength. Mostly the smaller units contain type 1 muscle fibers, whereas the larger units have type 2 representation. The deltoid muscle contains approximately 1000 motor units, 60 % of which have type 1 fibers. The first dorsal interosseus muscle has approximately 120 units, of which approximately 57 % contain type 1 fibers, but individual differences exist (Rothwell 1994). Motor activity of a muscle is recruited sequentially through the Henneman recruitment principle (Henneman and Mendell 1981), whereby small, slow motor units (containing type 1 muscle fibers) are activated before the larger and faster motor units containing phasic muscle fibers. The recruitment principle has been called the size principle of recruitment by Brodal (2001) and recruitment order by Rothwell (1994). The force of contraction may be graded in two ways (Brodal 2001): 1. The number of motor units that are recruited. If the number increases, so does the force production 2. An increase in the impulse frequency of the motoneuron, which also leads to increased force production Fig. 1.1 A motor unit is comprised of a number of muscle fibers with the same muscle fiber type, the α-motoneuron that innervates it, and the axonal branches of the α-motoneuron to the individual muscle fibers. Muscle fibers belonging to different motor units are dispersed within the muscle. Small motor units that demonstrate the greatest ability for endurance (“tonic activity,” sustained activity) occur in maximum numbers in muscles whose the main function is postural activity, i. e., sustained activity against gravity. Several authors have described postural activity as the basis for function of the extremities (Dietz 1992, Massion 1992, 1994, Shumway-Cook and Woollacott 2006). Motor units in a muscle are recruited sequentially, whereby the smaller motor units are activated before the larger motor units. Postural stability is the basis for selective movement control and function. Most muscles have a mixture of different motor units. The musculature is therefore able to function in relation to different activities: a muscle may have a stabilizing function in cooperation with some muscles or more of a mobility function when working with others. Motor units are recruited sequentially to enable the musculature to grade its activity in relation to strength, synergistic musculature, and required function (Massion 1992). Muscles can vary their activity and function as agonists, antagonists, or synergists depending on how they are being used. Most muscles have internal selectivity based on the distribution of motor units and muscle fiber type and size. Motor units may be activated differentially; some may work eccentrically at the same time as others are working concentrically to varying degrees. Anatomically defined muscles that cross two joints or more may excentrically lengthen over one joint while shortening over the other. This ability is called compartmentalization (van Ingen Schenau et al. 1990). Example The quadriceps muscle continuously varies its activity during locomotion; in stance phase, the proximal part has to contract eccentrically to allow for hip extension, whereas at the same time the distal part has to work concentrically to stabilize the knee for weightbearing. During the initial swing, the activity of the quadriceps is reversed; the proximal part undergoes more concentric contraction to assist in swinging the leg forward whereas the distal part works more eccentrically to allow knee flexion. Compartmentalization describes the ability of a muscle that crosses more than one joint to perform different functions simultaneously. Muscle balance is the result of cooperation within and between many muscles or muscle groups surrounding a joint: agonists, antagonists, and synergists. In humans with an intact CNS and musculoskeletal system the grading of activity in the different muscle groups is finely tuned and adapted to the relevant function and situation. Maintenance of muscle balance depends on neurologic, muscular, and biomechanical factors (Sahrmann 1992, 2002, see also Stokes 1998): • Muscular factors—such as the length–tension relationship of the muscle and its ability to produce force appropriately • Neurologic factors—the sequence of recruitment of motor units within the muscle and the sequence of activation of different muscles or muscle groups • Biomechanical factors—alignment, structure, and function of the joints Muscle imbalance may result if any of the abovementioned factors are disturbed, for instance neurologic problems leading to malalignment. Muscle balance depends on muscular, neurologic, and biomechanical factors. Alterations in recruitment and the distribution of motor activity affect alignment. Altered alignment affects muscle function. Zackowski et al. (2004) describe impaired joint individuation as the inability to fix joints that should have been fixed during movement of another joint, and explain this phenomenon as impairment in motor control. These authors refer to other studies providing further evidence for reduced capacity of an impaired limb to generate certain muscle co-activation patterns. This may be due to abnormal spatial tuning (distribution) of muscle activity. The connective tissue unites, supports, and holds the structures of the body together. Fibrous tissue is elastic and supports muscles and joints as well as allowing movement. With increasing age, the fibrous tissue loses its strength and elasticity. If fibrous tissue is kept shortened, contractures may result (Tyldesley and Grieve 1996). Muscle spindles are specialized receptor organs found in between and in parallel with muscle fibers in skeletal muscles. The muscle fibers of a muscle spindle are called intrafusal fibers. The muscle spindles inform the CNS of the length of the muscle, length changes, and the speed of change via primary (Ia) and secondary (II) afferent nerve fibers (Fig. 1.2). The muscle spindle is innervated by a γ-motoneuron, which can cause contraction and so alter the length of the spindle. Therefore it can modify the sensitivity of the spindle during alterations in length or stretch of the muscle. Muscle fibers are innervated by α-motoneurons. In a relaxed muscle there is hardly any detectable activity in the muscle spindles, and the probability of impulse activity in the γ-motoneurons is therefore small. The impulse frequency from the Ia afferent fibers increases strongly with isometric contraction of the muscle. This is caused by an increase of γ-activity as the α-motoneurons stimulate the extrafusal muscle fibers to contract. This is called α-γ co-activation. The sensitivity of the muscle spindle seems to increase when the muscle works actively. If a muscle actively contracts and shortens, the impulse frequency of the Ia fibers is maintained at a high level, which indicates that the γ-motoneurons also have to increase their activity (Brodal 2001). The function of the γ-motoneuron is to maintain the sensitivity of the muscle spindle during muscular activity (Kandel et al. 2000). This system seems to be important for the maintenance of tonic contractions, e. g., keeping the knee stable during loading of the leg in stance phase. The musculature depends on a harmonious interplay between afferent information from the spindles and efferent motoneuron activity. Through this, the muscle is always ready for alterations in activity. Fig. 1.2 The muscle spindle and the Golgi tendon organ. The muscle spindles are situated in between and in parallel with the extrafusal (skeletal) muscle fibers and are attached to the tendon through connective tissue. The number of muscle spindles in different muscles vary, with greater numbers present where the need for precise grading of activity is necessary e. g., in the small muscles of the hand or the deep postural muscles of the back. Golgi tendon organs are specialized receptors that inform the CNS about tension changes within the muscle (Brodal 2001). They are innervated by a sensory nerve fiber, the branches of which encircle the connective tissue fibers in the organ. The connective tissue fibers are attached to muscle fibers at one end and to the tendon proper at the other end (see Fig. 1.2). These muscle fibers belong to many different motor units. The tendon organ is therefore able to detect tension changes and the distribution of activity in different motor units within the muscle at the same time. Tone is related to the state of the muscle fibers, the activity within the sense organs, muscle viscosity, and connective tissue. Muscle tone is an expression of the stiffness of the tissue. The relationship between muscle length and tension is called stiffness (Brodal 2001). The control of this relationship is called stiffness control. Muscle tone is usually the term used to describe the tension in relaxed muscle, and is also called resting tone. Brodal (2001) states that the most important factor in changing the level of tone is muscular contraction. The viscoelastic properties of the muscle fibers, connective tissue in the muscle and the muscle tendon add to this to a lesser degree (see Simons and Mense 1998 for discussion). Shumway-Cook and Woollacott (2006) define postural tone as activity in muscles that counteract the force of gravity in the upright position. They state that “muscles throughout the body, not only those of the trunk, are tonically active to maintain the body in a narrowly confined vertical position during quiet stance.” They use the term ideal alignment to describe the increase of muscle work needed when the body moves outside a narrowly confined vertical position, i. e., even small ranges of movement increase the demands on muscular activity. To maintain normal function, tone needs to be high enough to allow the body to be dynamically active in relation to gravity. Postural tone is influenced by information from somatosensory receptors (skin receptors in the soles of the feet and neck receptors among others) and visual and vestibular input. Other factors that influence tone are pain, fear, and input from other areas of the brain and spinal cord. Tone is related to the state of the muscle fibers, the activity within the sense organs, muscle viscosity, and connective tissue. The most important cause of alteration of tone is muscular contraction. Information to the spinal cord comes from all somatosensory receptors in the body, for instance skin, joints, connective tissue, muscles, tendons as well as from other sensory systems (vision, hearing, equilibrium) and other motor systems within the CNS. On any one motoneuron there may be as many as 50 000 synapses from sense organs and receptors, and from all levels and pathways within the brain and spinal cord (Fig. 1.3). Information is modulated continuously, and may result in motor activity. Muscle length, tension, and activity will in most situations be appropriate for the function or activity to be performed because of this integration of information (Brodal 2001). Many people who have suffered a CNS lesion have a reduction in balance, selective control of movement and strength. The lesion itself, how the person is being positioned (how he sits, lies), demands for independence, stimulation, training, and the person’s ability to activate and control his own body, will influence the neuromuscular adaptations that occur over time. The neuromuscular system adapts to the new situation and how the body is being used. This may lead to: Fig. 1.3 The synaptic connections to the motoneuron. There may be as many as 50 000 synapses on one motoneuron. • Changes in the length–tension relationship, alteration of tone • Altered recruitment pattern of motor units, which may disrupt the ability to stabilize the body/body part as a background for movement • Muscle imbalance (reduced interplay between different muscle groups) • Muscle fiber type changes and the level and constitution of connective/fibrous tissue; increased or decreased length of muscles; the muscle may be too stiff or stretched and cannot be activated efficiently and functionally • Changes in the way the patient moves and uses his body; the need for new movement strategies to achieve goals. When the patient lacks the ability to initiate the appropriate activity for a required function he find other ways—compensatory strategies. Patients use the movement strategies available to them to be functional here and now. Inappropriate activity may strengthen the above factors and complicate and limit the patient’s choice of movement in both the short and the long term • Alignment problems as a result of tonal factors, use, changes in contractile and noncontractile tissue will affect muscle function • Altered somatosensory information or perception may affect the patient’s ability to move Hypotonia and hypertonia are terms used to describe tonic changes within muscle. Brodal (2001) describes hypotonia as reduced tone in the musculature. In a clinical situation, this is perceived as reduced ability to activate muscles appropriately and as a lower muscular tension or stiffness than expected in the same situation when compared with people without a CNS lesion. Hypertonia is described as a continuous increase in tension or stiffness even if the person attempts to relax (Brodal 2001). Clinically this is perceived as aninability to grade and modulate tone, or as a higher muscular tension or stiffness than expected in the same situation when compared with people without a CNS lesion. The musculature seems to lose its variability and flexibility in CNS lesions. Altered distribution of motor unit activity and changes in alignment may affect muscle function negatively—the muscles have new working conditions. The patient therefore may be unable to align and recruit the neuromuscular activity necessary to reach the desired goal efficiently. Efficient in this context means that the patient does not need to use more force or exertion than would be normal for a person without a CNS lesion. The latissimus dorsi is anatomically described as one muscle, but comprises many segments working together to create function. When all of the latissimus contracts, it brings about extension of the spine, increased lumbar lordosis combined with internal rotation, adduction, and extension of the arm. During normal conditions, the latissimus is able to activate differentially by increasing lumbar extension at the same time as the arms are stretched above the head, as a jumper is pulled over the neck and head while undressing (Fig. 1.4), i. e., it contracts concentrically distally and eccentrically in relation to arm function proximally—both at the same time. In my clinical experience with CNS lesions, it seems as if the ability of the muscle to perform differential actions is disturbed. The patient’s ability to compartmentalize muscle activity may be reduced, and the muscles seem to contract in their total range of movement when activated. In the case of the latissimus dorsi, the arm tends to rotate internally, adduct and extend together with lumbar extension when activated. If this total pattern of movement is learned, the patient’s functional ability and independence will be reduced. Fig. 1.4 Activation of the latissimus dorsi. Muscle fiber changes have been shown in CNS lesions (Ada and Canning 1990). Inactivity due to immobilization, denervation, or reduced activation makes the musculature prone to atrophy. Muscle fiber type changes may also be present: Hufschmidt and Mauritz (1985) state that a tonic transformation of muscle fibers may be one reason for the increased resistance to stretch experienced in spastic muscles. Phasic muscle fibers may be transformed to undergo more tonic activity. In many neurologic conditions it seems as if the patient’s postural control is mostly affected. Clinical experience suggests that the patient uses the strategies available to him to maintain balance, for instance by fixing with the arms, increasing arm support, or flexing and adducting the hips. When the arms are used for balance, they are being recruited to support the body and are not free for functional use. Normally, phasic activity dominates in arms due to the need for rapid movement in a variety of different contexts. When the arm muscles are recruited to maintain stability, the functional demands on arm musculature change, and a gradual transformation of muscle fiber type may ensue, contributing to the increased stiffness of arm musculature experienced by some patients. Muscle length changes occur in pathologic conditions of the CNS (Goldspink and Williams 1990). When patients are kept sitting for many hours each day, the hip flexors are kept in a shortened position. The muscle fibers shorten and adapt to the position in which they are being held. This may lead to a reduction of sarcomeres so the muscle becomes anatomically shortened. When the patient attempts to stand up or is being transferred through standing by helpers, the shortened muscles experience stretch. The muscle spindles and Golgi tendon organs inform the spinal cord of the stretch and tension, the α-motoneurons are activated to contract the hip flexors to take the tension off the spindles, and the hip flexors contract too early due to predisposed recruitment. So the patient either lifts the leg off the floor and is thereby destabilized, or is pulled down in the hips and pelvis and is not able to reach a standing position. Conversely, the patient’s hip extensors are in a lengthened position while sitting. As the patients may sit for many hours each day, the hip extensors are passively stretched and are stimulated to “grow” in length. As a result, the number of sarcomeres may increase, which leads to the muscle being unable to produce an appropriate amount of force to allow the patient to stand up, maintain standing, or stabilize the hip during the stance phase of walking. This is due to over-stretch weakness. As a result, the patient may have to use his arms for support during transfers, in standing, and walking. The use of arm support increases the patient’s flexor activity (pressing down) through the arms and trunk—the patient therefore attempts to maintain standing through flexor activity and negate extension. If a patient’s arm is kept positioned on a table in front of him or in his lap for long periods every day, there is a danger of the biceps shortening distally. Proximally the length changes will depend on the position of the shoulder. Triceps will experience prolonged stretch distally and usually shortening proximally. Both lose their ability to be activated functionally (Ada and Canning 1990). Inactivity causes the amount of fibrous tissue within the muscle to increase and the muscle becomes stiffer (Goldspink and Williams 1990). The opposing muscle groups, joint capsule, and ligaments may become stretched and the stiffness decreases in the stretched muscle. There may be, as a result, an imbalance in the supporting tissues and therefore loss of stability. This may negatively affect the patient’s ability to move. The term somatosensory is related to sensory experiences within the body (soma). In this chapter the discussion will be limited to sensory information from the skin, joints, and muscles. Somatosensory information is received and carried through the peripheral nervous system; from receptors in the body to the spinal cord. Somatosensory information is to some degree modulated and integrated in the spinal cord. Information is transmitted through ascending fibers that synapse with interneurons and moto-neurons at spinal level as they ascend to the brain. Somatosensory information is transmitted via the dorsal root ganglion of the spinal cord and two ascending pathways: the anterolateral system and the dorsal column medial lemniscus pathway (Fig. 1.5) (Kandel et al. 2000). • The anterolateral pathway comprises of the spinothalamic tract and the spinoreticular tract, which transmit information related to pain and pain/temperature, respectively. Information ascends contralaterally in the spinal cord to the thalamus. • The dorsal columns and the medial lemniscus pathway: the gracile fascicle and cuneate fascicle and the spinocerebellar tract. The fasciculi cuneatus and gracilis pass on information from receptors in the skin and joints in the upper and lower part of the body, respectively, which travel ipsilaterally to the cuneate and gracile nuclei in the brain stem. Here, they cross over to form the medial lemniscus. As the fibers ascend further, they gradually move more laterally and join fibers of the spinothalamic tract in the thalamus. Fig. 1.5 The figure illustrates the dorsal column medial lemniscus system—the sensory nerve entering the spinal cord, and the ipsilateral pathway to the nuclei cuneate and gracilis in the brain stem. Here the nerves cross over to form the medial lemniscus. The spino-reticular and the spinothalamic tracts cross over as they enter the spinal cord to form the anterolateral system. From the brain stem these two systems ascend together to the thalamus and to the cortex. (Redrawn with permission from Kandel et al., 2000, p. 447.) Somatosensory impulses are modulated in the thalamus, which also receives information from the basal ganglia, cerebellum, the eye, and auditory nuclei. Therefore it has an important role in making the cortex aware of sensation. The thalamus has connections to the basal ganglia, the cortex, the red nucleus, and cerebellum. As a result of this network, it is able to process different types of information and has a close relationship to motor and sensory areas of the brain and to the basal ganglia. Thus it has a role in interpreting the world. The somatosensory impulses leave the thalamus and travel to somatosensory areas of the cortex via the internal capsule. Direct pathways originate in the interneurons within the spinal cord. Two such important pathways are the ventral and dorsal spinocerebellar tracts, which terminate in the spinocerebellum as mossy fibers (see Cerebellum, page 34). They send proprioceptive information to the cerebellum from the legs—from joints and the activity within muscle, and from the muscle spindles and tendon organs, i. e., information about movement and about descending commands reaching the inter-neurons. Fig. 1.6 The sensory and motor homunculi show the somatotopic organization of sensory and motor representation within the cortex. Data indicate that loading (heel-strike) and unloading (heel-off) in locomotion provide the CNS information from spatial and temporal parameters, proprioceptive information, pressure receptors, and recruitment patterns of the lower limbs (Trew and Everett 1998). Weightbearing regulates the step cycle by influencing stance duration, and sensitivity to loading has been observed in humans. The degree of leg extensor activation is highly correlated with the percentage of body loading and has been found to be functionally phase dependent (Mudge and Rochester 2001). Data demonstrate that the level of loading through the limbs during cyclic activity can provide important information that facilitates the generation of steplike efferent patterns by the human lumbosacral spinal cord, by providing cues that modulate the activity of the motoneurons innervating the lower limb muscles. Limb unloading has been shown to be an important signal for the termination of stance and the initiation of swing in cat locomotion (Harkema et al. 1997). Heel-strike is important for initiation of stance and therefore locomotion. Heel-off is an important signal for the termination of stance, and therefore for swing phase. Patients with CNS lesions often have reduced control and mobility of the ankle and foot; at times hypertonicity or stiffness of the calf muscles prevents heel-strike, and the patient may show inactive dorsiflexion, or activates patterns of inversion and plantarflexion during walking. An inability to place the heel on the floor as the first component of stance or to lift the heel off the floor as a signal for the termination of stance disrupts the ability to achieve a stable stance phase of locomotion as a prerequisite for an efficient swing phase. More than half of the human cortex contains association areas that coordinate events arising in the motor and sensory parts of the CNS. These areas are involved in planning, thinking, feeling, perception, speech, learning, memory, emotions, and motor skills. Large parts of descending commands modulate sensory information in the spinal cord and brain stem. Sensory information modulates motor activity at all levels within the CNS. The primary sensory cortex (SI) has cortical representations of the different body parts, called cortical maps, and is the first level where humans perceive sensory information. This has been represented as the sensory and motor homunculi (Fig. 1.6). SI, SII, and the posterior parietal cortex (Fig. 1.7) all receive information from the thalamus. The sensory areas send information to both motor and association areas of the cortex, which is important for the ability to recognize and localize sensory stimuli. Fig. 1.7 The three major divisions of the somatosensory areas of the cortex: primary sensory cortex S-I, secondary somatosensory cortex S-II, and the posterior parietal cortex. Precise motor activity depends on close integration with the sensory systems. Most movements require a constant flow of information from receptors in the skin, joints, and muscles to evaluate if the movement is proceeding as planned. Information from the ocular and the vestibular systems can be of vital importance for motor performance. Sensory information enables the CNS to update and correct the outgoing commands to the musculature—either during ongoing movement or the next time it is being performed (Brodal 2001). Activity in ascending fibers may simultaneously affect the activity in the descending fibers, and vice versa. Somatosensory and visual information is crucial for exploration of the environment. Human interaction with the environment forms the basis for muscular activity, movement, and balance. Motor activity is context based. Visual information travels from the eyes to the visual cortex in the occipital lobe of the brain for awareness of visual input. Visual impulses are also transmitted to the nucleus superior colliculus in the brain stem, both directly from the optic nerve and indirectly through the visual cortex. Human beings are therefore able to automatically turn their head toward something in the environment and the control eye movements. Visual information is also transmitted to the cerebellum and has a role in coordination. Vision is essential for mobility, the ability to read, visual orientation, and activities of daily living (ADLs) (Kerty 2005). Visual information is important for anticipatory adjustments to movement, in feedforward motor control and eye–hand contact for precise manipulation of objects. The use of a knife and fork for eating or the ability to thread a fine needle depends on sensory and visual information that enables us to get food on the fork or the thread into the eye of the needle. If vision fails, other somatosensory inputs compensate. People without eyesight or reduced eyesight develop an increased sensitivity to specific receptors in the skin to be able to control movement with good precision. As we move about we receive visual information, both about the environment and the relative position and movement of the body within the environment. The eyes scan the environment in the direction of movement: the height of the steps on a staircase, the position of furniture or any obstacles, and the position and movement of people. Vision is important for appropriate placing of our feet if the terrain is unknown, uneven, or complex, or when balancing on a narrow beam or near the edge of a cliff, but it does not dictate the detailed placing of the foot during normal walking. The dependence on visual information reduces as the environment becomes more known. Fig. 1.8 Areas for vision, motor activity, speech, hearing, cognition, somatosensation, and emotion within the brain. Many of these areas receive or integrate information from the visual system. The numbers refer to Brodmann areas, which are histologically defined regions. (Reprinted with permission from Professor Mark W. Dubin, MCD Biology, University of Colorado at Boulder.) If vision fails, as when moving through a dark room, then proprioceptive and vestibular inputs become more important for movement. On the other hand, vision may compensate if information from other systems fails, and may become the main contributor in balance control. The interaction of the different systems involved in movement and balance are adaptable to new conditions by weighing the relative importance of the different afferent systems in relation to what is needed (Brodal 2001, Wade and Jones 1997). Visual problems are present in as many as 40 % of people who have had a stroke and in 50 % after traumatic brain injury (Kerty 2005). Visual disturbances following a stroke are a result of the integrative nature of the brain and the fact that visual function involves considerable areas of the brain (Fig. 1.8). The one-sided visual defect often seen in stroke (hemianopia) is cause by a lesion to the optic nerve tract from the optic chiasma to the occipital cortex. A stroke may also affect association areas in the lower temporal region (recognition of objects or shapes) or the posterior parietal cortex (spatial awareness) (Riise et al. 2005). Visual defects may therefore be a contributor to a patient’s balance and functional problems. Ascending and descending systems are closely linked both anatomically and functionally. It is therefore inappropriate to discuss these separately. Motor activity is the result of a complex interaction between sensory, motor, and cognitive systems. The terms balance and postural control are often used interchangeably (Chapter 2 discusses these terms in greater depth). Reduced postural control is recognized as a major factor contributing to mobility problems in stroke patients. It is caused by dysfunction in the complex interaction of motor, sensory, and cognitive processes (de Haart et al. 2004). Balance is affected by afferent visual, vestibular, and proprioceptive information. Which system is weighted most depends on the need of the moment, and the relationship between the individual, the environment, and the function to be performed. Our CNS needs to be aware of where the body is, where the different body parts are in relation to each other and the environment, and the activity within the different regions of the body to be able to respond appropriately. Vestibular information seems to be dealt with in a distributed cortical network. The integration of other signals, spatial information, body position, and movement occurs within the same network. This is connected to other cortical networks, for instance motor networks, and vestibular information probably contributes to our conscious awareness of body position and movement in relation to the environment (Brodal 2004). There is a continuous demand for adaptation to the environment, and therefore for variation in stability, balance, and movement. We move in relation to our base of support, and in standing or walking, our feet must adjust to the floor, the grass, stones, or sand. Several studies demonstrate a close relationship between anticipatory adjustments (feedfor-ward), associated adjustments (ongoing adjustments), and adaptations (feedback) in normal postural control (Horak et al. 1997, Wade and Jones 1997). “The classic concepts that the movement originates and commences in MI [primary motor cortex] and that the PT [pyramidal tract] represents the highest level of motor control are now thought to be incorrect. The modulation of PT neuron firing is determined largely by the 3 major types of information that MI receives and processes: internal feedback and external sensory feedback “ (Davidoff 1990, p. 334). • Anticipatory adjustment of muscles (feedfor-ward) is an integral part of every movement, and is called central motor programming. • Anticipatory adjustment depends on internal feedback about the position of different body parts to each other, their activity and alignment, the relationship between the body and the environment, and the function to allow for error correction before errors occur. Anticipatory adjustment of alignment and muscular activity are therefore dependent on somato-sensory feedback. • As movement is being initiated, the CNS receives continuous impulses from receptor organs (eyes, muscle spindles, tendon organs, joint receptors, pressure receptors, skin receptors) and can correct movement as required. This is a feedback function, external sensory feedback, whereby planned and performed movement may be compared and differences corrected. This is especially important for precise, small movements and postural stability. Anticipatory adjustments of muscle activity (feedforward) are dependent on both external and internal feedback. That is, information about the internal relationship between body parts, the relationship between the body and the environment and from specific receptors from the eyes, muscle spindles, golgi tendon organs, and receptors in the skin is necessary for feedforward and therefore for balance. Through feedforward the CNS may set an appropriate level of motor activity as a preparation for movement. Wade and Jones (1997) describe anticipatory and associated adjustments together as postural adjustments closely connected with the performance of voluntary activity. There is a belief that these types of adjustments reduce the postural displacements associated with a displacement of the centre of gravity caused by voluntary movement, as well as affecting voluntary movement directly. Massion (1992) states that motor activity may be recruited sequentially (after each other) or in parallel (simultaneously): • Either first the anticipatory activity, until the postural adaptations eliminate the displacements caused by movement and thereby stabilize the body, and then movement of arms or legs in standing • Or simultaneous (parallel) activation of stability and movement The most important component of the human ability to stay upright is continuous information about the effect of gravity through specific receptors, for instance proprioceptive receptors registering weightbearing, the distribution of pressure, and the line of gravity in relation to the feet. Dietz (1992) stated that postural activity depends on the activity in specific receptors informing the CNS of deviations in the center of gravity from a certain neutral position. This is what Shumway-Cook and Woollacott (2006) call the ideal alignment. Pressure receptors are distributed throughout the body, in joints and the spinal column as well as the soles of the feet (Petersen et al. 1995, Brodal 2001). Studies indicate that there are specialized gravity-dependent receptors in our internal organs also (Karnath et al. 2000). Activity in postural muscles, such as the soleus (lower leg), also seems to be important in this respect. If specific receptors are to inform the CNS of displacement, they have to be fired by activation and variation in the sensory impulses through movement, alteration of pressure areas, changes to the stiffness–tension relationship within muscle, and alteration of joint alignment. Mobility of joints and soft tissue is important to fire the sensory receptors and therefore for balance. Mobility combined with good alignment and appropriate neuromuscular activation gives the patient the ability to respond to displacements. Information (feedback) about muscle activity, movement, alignment, and weight distribution is important for balance, postural adaptation, and function. Mobility is a prerequisite for the firing of specific receptors in relation to displacements and variations in sensory input, and therefore for balance. We need information from the somatosensory system about the position of our body in the environment to plan and perform functional movement. An updated body schema is a prerequisite for appropriate and efficient feedforward. When standing on a stable base of support, somatosensory information is especially important to generate automatic postural adjustments and provides the most important, although not the only, input to postural control (Nashner 1982). Dietz (1992) states that all motor activity in standing or walking both starts and finishes with postural adjustments. Mulder et al. (1996) says that the ability to react needs a higher level of sensorimotor (re) integration than anticipation. An updated body schema is a prerequisite for appropriate and efficient feedforward control. Stereognosis is described as “The faculty of perceiving and understanding the form and nature of objects by the sense of touch,” that is, without the use of vision (Mosby’s Medical, Nursing and Allied Health Dictionary 1994). Stereognostic sense relates mainly to hand function. The stability, mobility, sensitivity, and adaptive ability of the hands are crucial for exploration of the environment and object manipulation. Stereognostic sense is a combination of: • The ability to move • Somatosensory receptors informing the CNS of variations • The ability to recognize variations (for manipulatory purposes) • Joint position sense (the net information from joints, muscles, tendons, and skin) • Perception Stereognostic sense is based on somatosensory information, movement, the ability to recognize variations and perception. There are several types of specialized receptor within the skin and epidermis, both free and encapsulated nerve endings that send different types of information to the CNS. Some of these receptors adapt quickly, others more slowly; some have a low threshold for firing, and others a high threshold. Fast -adapting means that the receptors quickly get used to the stimulus and stop firing even if the stimulus continues. This type of receptor informs the CNS of change, the start and finish of impulse firing, i. e., variations, and reacts to touch, pressure, and stretch. Other types of receptor are slow -adapting and continue to send information as long as stimuli are present. In this way, the CNS is updated about the state of the body at all times. Examples include information from muscle spindles and tendon organs. Nociceptors sensitize, i.e., their sensitivity increases with continuous stimulation. A quietly dripping tap becomes an unbearable noise after some time. If water drips on the head, the individual water droplets will feel like the blow of a hammer after a relatively short time. Some people react to wool directly on the skin with irritation or a rash. Discomfort or pain may be amplified over time until it occupies all thought. The adaptive ability of the CNS allows us to wear clothes without our brain becoming over-bombarded with stimuli from all the specific receptors. Adaptation protects the CNS and modulates incoming information. Some different receptor types are listed below (from Kandel et al. 2000, Brodal 2001): • Free nerve endings—high-threshold mechanoreceptors (nociceptors) • Meissner corpuscles—fast-adapting, low-threshold (light touch, e. g., fly crawling on the arm) • Pacinian corpuscle—fast-adapting (vibration) • Ruffini terminals—slow-adapting (friction, stretch of skin) • Merkel disks—slow-adapting (continuous pressure, especially in distal areas of the extremities, lips, and the external genital organs) Somatosensory information in the cortex is somatotopically arranged, representing different areas of the body (see Fig. 1.6). For somatotopic mapping of cortical function, it suffices to know which neurons respond to a stimulus at a particular site on the body. Each part of the body is represented in the brain in proportion to its relative importance to sensory perception. The map represents the sensory innervation density of the skin rather than its surface area. A sensory unit is a sensory nerve cell and all its branches, in the body and the CNS, and a receptive field is defined as the area of the body from which the sensory unit receives stimulation. Two-point discrimination is the result of two receptive fields being stimulated at the same time; each point must stimulate a sensory unit to enable the CNS to feel them both. The skin of the fingertips is the most densely innervated area of the body with approximately 300 mechanoreceptive nerve endings/cm2; on the proximal phalanges there are 120/cm2, and in the palm of the hand 50/cm2. This means that two-point discrimination varies between the fingers and hand: it is approximately 2 mm on the finger tips, 10 mm in the palm of the hand, and 40 mm on the arm (Kandel et al. 2000). Fig. 1.9 Lateral Inhibition increases the contrast of sensory impulses. Afferent information is modulated and localized through lateral inhibition (Rothwell 1994, Kandel et al. 2000, Brodal 2001), especially in relation to the fast-adapting receptors. When skin is stimulated, information is sent to the CNS. Where the stimulus is at its strongest, for instance at the edges of a book being held in the hand, the stimulated receptor organs transmit impulses to the spinal cord, where they give off collaterals that synapse with inhibitory inter-neurons in the dorsal horn (Fig. 1.9). The inhibitory interneurons inhibit sensory impulses from other sensory neurons arising from the periphery of the receptive field of the stimulated area. Therefore the sensory units in the center of the stimulated area are stimulated the most. Thus the CNS is able to localize touch and is informed of changes (start/stop), edges, texture, and shapes, i. e., variations. If a person places his hand on another person’s shoulder, he is informed of the shape and temperature of the area. If the hand is not moved, the receptors quickly adapt and stop sending impulses. So without moving the hand, the size, shape, texture, and temperature of an object cannot be evaluated. Movement is necessary to activate receptors and obtain information through variations, and therefore for sensation. Motor activity and sensation are closely linked, and motor activity is a tool of sensation. Brodal (2001) states that most movements need continuous information from specific receptors within muscles, tendons, joints, and skin to know if the movement is progressing as planned. The ability of the neuromuscular system to regulate tension and length (stiffness control) combined with information from joint and skin receptors provides the CNS with precise information about the position of joints and therefore of the position of body segments relative to each other. If the joint mechanoreceptors are anesthetized, the brain still receives precise information about joint position through muscle activity—muscle spindles and tendon organs—and skin receptors (Brodal 2001). The Golgi tendon organ is stimulated more through active contraction of muscle than through passive movement. Brodal (2001) states that joint position sense is the conscious awareness of joint position, of movements of joints, and of the direction and speed of movement (without visual information). Joint position sense is the perceived result of sensory stimuli from proprioceptors and skin. Muscle activity is important for joint position sense. The brain stem contains many specialized neuronal groups (nuclei) that react to new sensory stimuli and increase the response of the moto-neurons to specific stimuli. These nuclei receive information from the spinal cord, i. e., from peripheral receptors. Through their connection to the cortex, the hypothalamus, limbic structures, and hippocampus and other areas, they cause increased activation, arousal, and awareness to the stimuli. Shumway-Cook and Woollacott (2006) state that “Perception is essential to action, just as action is essential to perception.” Human beings need to be able to receive and integrate information from stimuli, and recognize, compare, and perceive stimuli to interpret what the information means. Variation in somatosensory information increases awareness of the stimulated body part. Somatosensory perception may change over time. The somatotopic maps are not fixed but can be altered by experience. The proportion of neurons in the cortex devoted to a specific area of the body will alter with use (Kandel et al. 2000). “If you don’t use it, you lose it” (Kidd et al. 1992). Studies on stroke patients have shown that sensory and motor stimulation significantly improves both somatosensory information processing and motor control (Sunderland et al. 1992, Yekuitiel and Guttman 1993). Wade and Jones (1997) state that being active in relation to the environment improves perception. The term learned nonuse refers to conditions in which reduced motor control leads to inactivity, e. g., reduced control of the affected arm in hemiplegia may lead to compensatory strategies whereby a patient uses his “good” arm and not the affected one. The affected arm is therefore not used, not stimulated and is inactivated. This predisposes to secondary muscular and soft tissue changes (Ada and Canning 1990), which may be important if the patient has reduced awareness or neglects the affected side. If the patient is not able to move, he receives little or no information from the receptors within skin, muscle, and joints; the ability to recognize changes in joint position may be reduced as a result. Through formal testing of joint position sense, only the patient’s perception and cognitive awareness are tested and not his possible potential for integration of joint position information through movement. Information from receptors is transmitted from the receptor areas in the body through to the spinal cord, modulated and passed on for further processing in the brain stem, the cerebellum, and the thalamus. Decreased sensory awareness will, for most patients with CNS lesions, be linked either to dysfunction in perceptual processing or to lesions in the ascending pathways within the brain itself, for instance at the level of the internal capsule. Therefore, somatosensory information will be integrated at many levels, even if the patient is unable to feel and perceive it. Only through observation of the patient in functional activity will the clinician be able to get a true picture of his ability to receive and integrate sensory information. Some patients with neurologic deficits have fixed stereotypical patterns of movement or may be unable to move a limb. Reduced movement and mobility reduce the stimulation and firing of receptors, and the CNS receives less information about variations in sensory information due to movement and the need for segmental adjustments. A consequence may be that the patient becomes more dependent on visual and vestibular information to maintain balance. In some patients the head and neck assume an asymmetric position due to imbalance between muscle activity and stability. Visual input may be distorted, and the firing of vestibular organs will be affected. This may cause further balance problems. Educating the patient about the position and control of head and neck posture is an important short-term goal in the rehabilitation of postural control. Other patients seem to visually override proprioceptive and vestibular information and the patient loses or reduces his ability to “listen” to his own body. The patient’s response to the environment and to displacements becomes more cognitive and less automatic. As a result, movement strategies change and tempo will be reduced. Interesting and varied sensory information through movement and stimulation may motivate and focus patients toward the stimulated body part or limb. It becomes important for the therapist to find which stimulus creates arousal and motivation of the patient’s CNS. The spinal cord receives information both from higher centers and from the periphery. It has a huge receptor area and both receives and modulates information from the whole body except the head before the information is transmitted to other systems or translated into muscle activity. It has a gate-control function whereby information sent to the spinal cord is adapted to suit the needs of the organism and to protect the brain from overstimulation (Davidoff 1990, Kandel et al. 2000). Because of its large receptor area and gate-control function, the spinal cord influences activity in the higher centers. “The existence of networks of nerve cells producing specific, rhythmic movements, without conscious effort and without the aid of peripheral, afferent feedback, is indisputable in a large number of vertebrates” (MacKay-Lyons 2002). Neural networks in the spinal cord, called central pattern generators (CPGs) are capable of producing rhythmical movements (Dietz 1992, Brodal 2001, MacKay-Lyons 2002). CPGs provide automatic, changing activity coordinating the two halves of the body and have been mostly studied in vertebrates. CPGs have been demonstrated for vital functions such as breathing, chewing, and swallowing and are located in the brain stem, whereas those for locomotive functions are contained in the spinal cord. The probability of their existence in humans is high. Evidence is emerging from research on patients with spinal cord lesions and others. A baby’s early stepping-reactions of rhythmical spinal activity may also be an expression of pattern generation. Fig. 1.10 A longitudinal view of the brain and spinal cord. The spinal cord and brain stem contain specialized groups of cells—neuronal pools called central pattern generators. In relation to reaching and grasping, Paillard (1990) states “Elaborate motor patterns are produced at the brainstem level by pre-wired circuitry. These circuits are triggered to operate at the required time and are automatically adjusted to postural requirements and environmental constraints through the servo-assistance of internal and external feedback loops at the spinal and midbrain level.” In humans, findings are consistent with separate CPGs controlling each limb (Dietz et al. 1994 in MacKay-Lyons 2002). These are linked together through a complex interneuronal network. The dorsal root fibers from peripheral receptors terminate on interneurons that branch within the spinal cord. Interneurons that spread their branches over several segments are called propriospinal fibers and are found throughout the white matter of the spinal cord (Brodal 2001). The impulses from one dorsal root-fiber may therefore spread over several segments both up and down. Long propriospinal fibers (from the cervical to the lumbar areas) are necessary for coordination between the right and left side of the body during walking, and rhythmical interchange of activity between the two sides is caused by cells with pacemaker properties. Propriospinal fibers also have a role in the transmission of descending motor commands (Rothwell 1994). The CPGs are, in their original form, independent of somatosensory information. Studies in humans and monkeys have shown that voluntary motor tasks, such as reaching and grasping, and more rhythmical movements, including swimming and walking, can be performed following deafferentation (Knapp et al. 1963, Rothwell et al. 1982 and Marsden et al. 1984 in MacKay-Lyons 2002). However, the interplay between central and sensory influences is critical in the production of adaptive behavior, i. e., for function. Somatosensory feedback is an integral part of the overall motor control system and is essential in modifying CPG-generated motor programs to facilitate constant adaptations to the environment (MacKay-Lyons 2002). The CPGs use afferent information from several sources in the visual, vestibular, and somatosensory systems. Afferent information is essential for adaptation of CPG activity for locomotion to make walking appropriate to the environment and to the reason for walking, i. e., to the external and internal environment (Pearson 1993): • Timing function: sensory feedback provides information ensuring that motor activity is adapted to the biomechanical state of the moving body parts for position, direction, and strength • Facilitation of phase-changes in rhythmical movements to ensure that the next phase is not initiated before the biomechanical state of the body is ready Dietz and Duysens (2000) state that afferent information is necessary to strengthen CPG activity of antigravity muscles. Information about unloading, heel-strike, and weight transference are critical for the control of stepping (Maki and McIlroy 1997). Kavounoudias et al. (1998) researched the role of cutaneous receptors in the soles of the feet for balance. They anesthetized the feet and found that subjects were unable to balance on one leg after the loss of foot sensitivity. A specific distribution of mechanoreceptors in the feet codes the spatial origin, the amplitude and the rate of changes in the amplitude of pressure exerted on the skin. Therefore the CNS is continuously receiving information regarding the spatial and sequential distribution of pressure from the soles of the feet. There is co-processing of multiple tactile messages within the foot and between the feet. The higher centers involved in the initiation, modulation, and control of locomotion are: • The midbrain locomotor areas • The vestibular system • The reticular formation • The cerebellum • The cortex From lampreys to primates, nuclei in the mesencephalon, referred to as the mesencephalic loco-motor region ( MLR) or the mid-brain locomotor areas, initiate locomotion through activation of the lower brain stem reticulospinal neurons (MacKay-Lyons 2002). Three different zonal areas have been identified, which all have a role in the initiation of locomotion for different reasons: the lateral hypothalamus initiates gait in relation to hunger, thirst, or the need for the bathroom, the zona incerta for tourist-type walking, i. e., visually directed walking, and the periventricular zone for anger and fear. The reticular formation and vestibular system in the brain stem are both involved in activating the antigravity musculature. Further modification is through the cerebellum, which is thought to coordinate the activity of the CPGs for the right and the left side of the body. The cerebellum is active in motor learning and error correction. The cortex exerts little influence on simple unobstructed walking, but has a role in visual scanning, perception, navigation, and in modifying the activity of the CPGs to make it appropriate to the moment: as walking becomes more complex, the cortex becomes more active. When locomotion becomes complex, especially where footfall is narrowly specified and the need for visual input increased, the cortex fires more rhythmically to guide the placing of the foot. The CPGs cannot see the ground. Walking on a flat, even surface is probably controlled by CPG in the spinal cord and brain stem and coordinated by the cerebellum, i. e., it is mainly an automatic activity. Pattern generation may have an important role in the early activation of postural activity and coordination in the acute/subacute stage in patients who have a CNS lesion. Facilitation of stepping in a simple noncomplex environment does not require cognitive problem solving by the patient: • A pattern-generated step is a result of selective displacement and is different in its motor activity from a reactive step resulting from over-displacement. • Pattern generation may be facilitated automatically, even in patents with severe motor, sensory, or perceptual dysfunctions. • Pattern generation may enhance motor activity in the body as a whole by facilitating the interplay and coordination between the different segments and the two halves of the body, thereby promoting balance control. • There are CPGs for each side of the body. A good stance phase resulting from heel-strike facilitates the swinging phase on the same side (release of kinetic energy). • A good stance phase provides stability to the postural system, and therefore also frees the opposite leg for swing. • Pattern generation depends on appropriate afferent information to adapt the activity to the environment. • Tempo must be at an appropriate level for the individual to facilitate phase changes. Individuals seem to have different inherent speeds. • Early facilitation of stepping facilitates the patient’s awareness of the environment, and may improve perception. Descending pathways from the brain stem and higher centers have executive functions and a role in modifying and modulating incoming information. They regulate the transmission of sensory information from the spinal cord to the brain, and affect the excitability in spinal inter-neurons at the same time as being responsible for motor activity (Rothwell 1994). They are dependent on somatosensory information to be up to date on the state of the body at all times. Descending pathways may be grossly divided into the lateral system and the medial system (Kandel et al. 2000). The medial system provides the basic postural control, based on which the cortical motor areas can organize more complex and differentiated movement. It includes the vestibulospinal tracts (medial and lateral), the reticulospinal tracts (medial and lateral) and the tectospinal tracts. These pathways descend ipsilaterally in the anterior (ventral) part of the spinal cord and terminate predominantly on interneurons and long propriospinal neurons in the spinal cord. Thus they influence motoneurons that innervate proximal and axial musculature. They also terminate directly on some motoneurons that innervate axial muscles. The vestibular system consists of several (four on each side) nuclei at the base of the brain stem (Fig. 1.11). These receive information from: • The vestibular organs in the inner ear, signaling the position of the head and changes in movement, direction, and speed • The spinal cord (proprioceptive information), reticular formation, the cerebellum and some nuclei in the mesencephalon (mid-brain, part of the brain stem), but not from the cortex directly • The eyes The vestibular system sends information to: • The spinal cord, especially to motoneurons • Brain stem nuclei for external eye muscles • The cerebellum The main function of the vestibular system is the control of balance in close cooperation with the proprioceptive and visual systems. The lateral and medial vestibular nuclei control posture. The lateral vestibular nucleus (Deiter’s nucleus) is concerned with control of the position of the head and body in relation to gravity. The lateral vestibulospinal tract extends the total length of the spinal cord, and affects α- and γ-motoneurons monosynaptically, and antigravity musculature (Markham 1987, Brodal 2001). Due to its monosynaptic connection, it reacts quickly to restore balance. The activity of the vestibular system is greatest in standing and walking where the demands on postural control are greatest. Any displacement of position or posture, weight transference, or movements of the arm displaces the center of gravity in relation to the feet and the base of support. Irrespective of how small the displacements are (breathing causes small, almost invisible alterations in the intersegmental alignment of the trunk, which are perceived as small perturbations to the center of gravity), muscle tone and activity will need to adapt to maintain equilibrium. The interplay between the body and the environment is crucial in standing, balancing, and walking. Somatosensory and vestibular information contribute to the perception of the body in space (Wade and Jones 1997). Fig. 1.11 The vestibular nuclei in the brain stem. The figure shows the most important afferent and efferent pathways. The activity of the vestibular system is greatest when postural control is required.
1.1 Systems Control: Systems and Structures Concerned with Movement and Sensorimotor Integration
The Neuromuscular System
Structure and Function of Skeletal Muscle
Skeletal Muscle Fibers
Functional Relevance
Muscle Balance
Muscle Imbalance
Noncontractile Elements
Sense Organs in Muscle
Muscle Spindles
Golgi tendon organs
Muscle Tone
Clinical Relevance
The Somatosensory System, Vision, and Balance
The Somatosensory System
Clinical Relevance
Sensorimotor Integration
Vision
Balance
Stereognostic Sense
Clinical Relevance
The Brain and Spinal Cord
The Spinal Cord
Central Pattern Generators
Clinical Relevance
Descending Systems
The Brain Stem
The Vestibular System and Balance
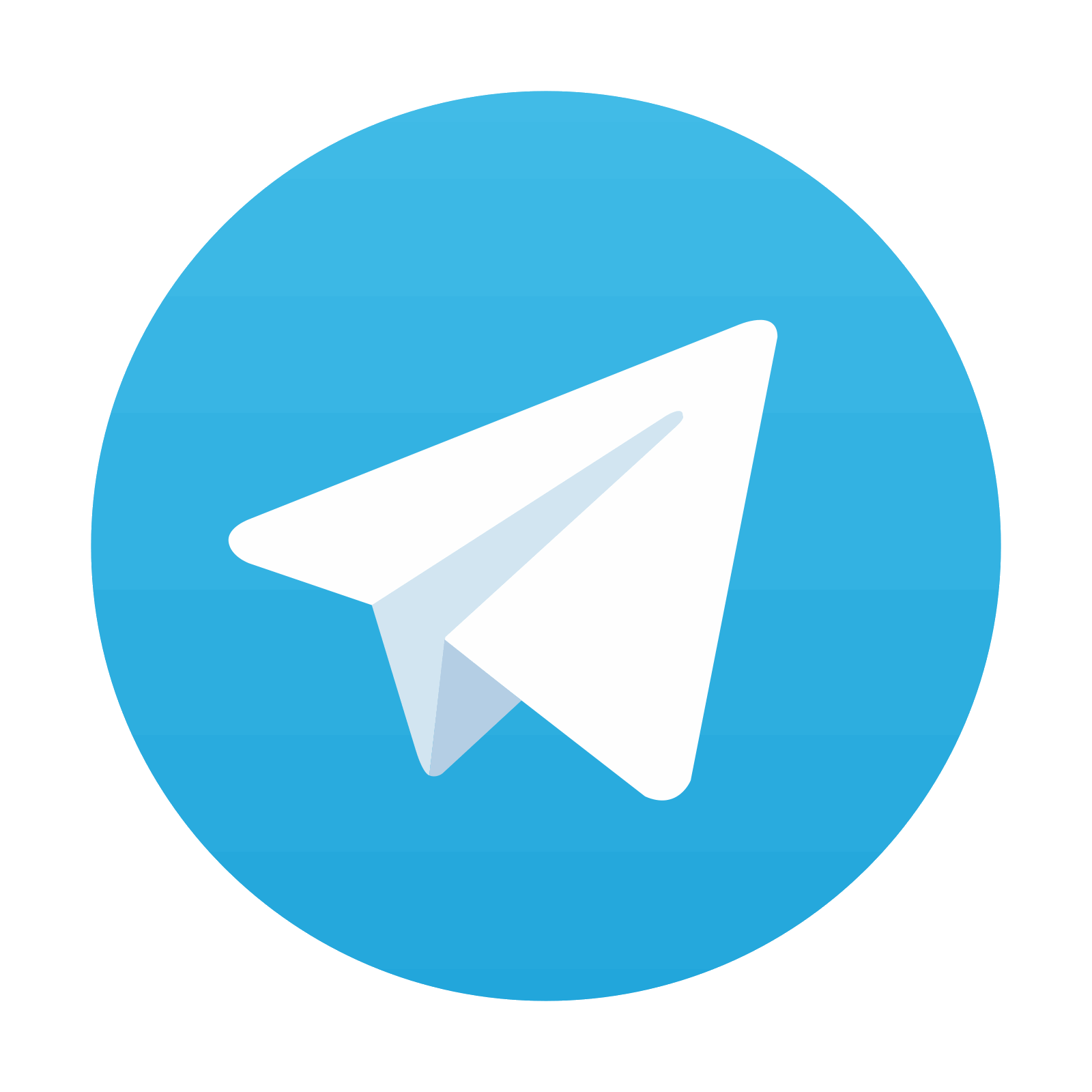