Fig. 1
REM sleep parameters plotted as 20 h totals for baseline (Base), two shock training days (ST1, ST2), and context (Con) in a study comparing the effects of controllable (modeled by escapable shock or ES) and uncontrollable (modeled by inescapable shock or IS) stress. a Total REM sleep. b REM sleep percentage (total REM sleep time/total sleep time). c Number of REM sleep episodes. d REM sleep episode duration. Significant differences between ES and IS: **, p < 0.01; ***, p < 0.001; ****, p < 0.0001 (Tukey test). Significant differences compared to Base (open symbols) are indicated by dark circles or squares for the ES and IS groups. Reprinted with permission from (Sanford et al. 2010)
3.2 Stressor Predictability
Predictability is an important factor in the effects of stress and a preference for predictability has been repeatedly demonstrated (French et al. 1972; Gliner 1972; Miller et al. 1974). For example, animals given the opportunity to determine whether shocks delivered to them will be signaled or unsignaled typically choose to spend their time in the signaled conditions regardless of whether the shock is escapable or inescapable [reviewed in (Badia et al. 1979)]. The strong behavioral effects suggest that predictability may also have a role in the modulating effects of stress on sleep. In fact, stressor predictability is a significant component in shock avoidance training in a shuttlebox, a paradigm in which laboratory rats are signaled of imminent shock and can learn to prevent shock from being delivered. Variants of this paradigm have often been used in studies of learning and sleep and have typically found increases in the amount of REM sleep at various latencies after training that have been interpreted as indicating a role for REM sleep in memory consolidation (e.g. Smith and Lapp 1986; Datta 2000). Unfortunately, the potential role of predictability in modulating sleep after stress has received very little attention.
One study of stressor predictability in mice examined sleep after training with signaled escapable shock (SES) and signaled inescapable shock (SIS) (Yang et al. 2011a). Compared to mice experiencing SIS, those experiencing SES showed significantly increased REM sleep after each of two shock training sessions whereas compared to mice experiencing SES, those experiencing SIS showed significantly increased NREM sleep after both shock sessions. Interestingly, groups receiving either SES or SIS showed reduced REM sleep in response to cue presentation alone. In another study, mice exposed to either predictive or non-predictive auditory cues during training with ES also showed post-stress increases in REM sleep (Machida et al. 2013). However, a subgroup of mice (around 35 %) trained with the predictive auditory cue failed to improve their escape performance from the first to second day of training. Those mice that did not improve also did not show enhanced REM on either shock training day, suggesting a learning component in the alterations in REM sleep.
It is useful to compare these results to those obtained using non-signaled escapable and IS used to model controllable and uncontrollable stress as described above (Sanford et al. 2010). Without predictive cues, the relative differences in post-stress REM after escapable and IS were more pronounced. Contexts associated with non-signaled escapable and IS also produced directionally different changes in REM similar to those seen when shock was presented (Sanford et al. 2010), whereas predictive cues associated with either escapable or IS produced similar reductions in REM sleep.
While at this point the data are limited, these findings suggest that contexts and auditory cues associated with different shock training conditions may carry different, and potentially competing, types of information regarding the stressful situations. This difference is more pronounced in training with ESs as both contextual and cued fear associated with uncontrollable stress have similar effects on sleep in mice and both reduce REM (Sanford et al. 2003a, c). Thus, competing cued and contextual information associated with ESs may have interacted during training resulting in competing influences on REM, thereby suggesting that stressor predictability and controllability may interact in complex ways to modulate the changes in subsequent sleep.
3.3 Stress-Related Learning and Sleep
In addition to producing direct physiological effects, stressful situations provide an opportunity for learning as the individual responds to the stressor and seeks to use available information to cope with the ongoing challenge the stressor imposes. While in humans this could involve a variety of activities, including higher order cognitive processing; in rodents, the simplest behavioral responses to a stressor may be avoidance or escape attempts. In this case, stress-related parameters such as controllability and predictability may provide useful information that shapes avoidance and escape behaviors thereby facilitating successful coping. By comparison, stressors that are uncontrollable or occur unpredictably do not provide information that can guide the animal to learn successful avoidance or escape behaviors. In these situations, the animal may still engage in escape attempts, but its behavior will not alter the presentation of the stressor or facilitate coping.
The impact of stressor controllability and predictability on behavior are central to a number of well-established learning paradigms that are motivated by stressful events. Of these, fear conditioning and related paradigms are beginning to demonstrate that the learning options an animal has in a stressful situation play a significant role in determining the impact of stress.
Experimental fear conditioning is a learning paradigm in which an animal makes an association between an uncontrollable stressor (usually footshock) and previously neutral cues (typically auditory) or contextual information (the test box and experimental room). Afterwards, presenting the fearful cues and contexts alone elicit physiological and behavioral fear responses similar to those produced by the initial uncontrollable stressor. Fear conditioned alterations in sleep are now also established though these can vary with the amount of training and with the strain of rats or mice that is studied. In agreement with the data in previous sections showing that uncontrollable footshocks reduce REM sleep, the primary and most consistent effect of extensive training with inescapable footshock is a marked reduction in REM sleep that occurs both after the shock training and after presentation of shock associated fearful cues and contexts (Sanford et al. 2003a, c; Tang et al. 2005d). This reduction in REM sleep has been reported across species and across strains (Sanford et al. 2001, 2003a, c) and can occur without the rebound or recovery REM sleep that has been reported for most stressors (Sanford et al. 2003a, c). Changes in NREM sleep in fear conditioning studies appear to be less consistent. Some studies have reported increases in (light) NREM sleep (Adrien et al. 1991), whereas others have shown strain-dependent reductions in NREM sleep after shock training and fearful contexts (Sanford et al. 2003a). There also may be relatively less NREM sleep EEG delta power (slow wave activity) in animals that show greater fear conditioned changes in sleep (Tang et al. 2006). Critically, these studies demonstrate that fear conditioned memories of stressful events can produce mostly the same changes in sleep as those produced by the stressful event itself and indicate the importance of learning in both the immediate and lasting effects that stress can have on sleep.
3.4 Fear Extinction and Sleep
Fear extinction is another important type of stress-related learning. While fear conditioning can be involved in the long term, negative effects of stress, it also can underlie adaptive behavior that occurs only so long as the fear-inducing stimulus is predictive of, or associated with, an aversive event (Kishimoto et al. 2000; Pitman et al. 2001). Repeated presentation of a fearful cue or context without shock results in fear extinction, a type of new learning that inhibits subsequent fear behavior without erasing the original memory for fear conditioning (Bouton 2004). It is the failure of extinction that has been linked to stress-related psychopathology, particularly posttraumatic stress disorder (PTSD) (Myers and Davis 2007).
The processes that make fear behaviors resistant to extinction remain mostly unknown though there appears to be a relationship between fear extinction and post-training REM sleep. Post-training REM sleep deprivation has been reported to impair extinction (as indicated by freezing) for light cues (Silvestri 2005), but not for auditory cues (Fu et al. 2007) previously paired with shock. REM sleep-deprived rats did show greater spontaneous recovery of freezing on a second day with presentation of the fearful auditory cue alone. Neither of these studies found that post-training REM sleep deprivation significantly altered contextual fear extinction learning or spontaneous recovery of freezing on a second day of testing (Silvestri 2005; Fu et al. 2007). However, sleep (both NREM and REM) following extinction of contextual fear does return to normal, whereas rats that continued to show fear exhibited reductions in REM sleep (Wellman et al. 2008).
3.5 Stressor Resilience and Vulnerability to Sleep Disturbance
Genetic differences in vulnerability and resilience are recognized as important factors in the development of stress-related pathology. For example, approximately 20–30 % of individuals who experience traumatic events may develop PTSD whereas others do not appear to suffer significant long-lasting effects (Cohen et al. 2003; Kerns et al. 2004). Attempts to develop animal models that better represent individual differences in clinical populations have included the selection of low and high responders to stressors in outbred rat strains (Cohen et al. 2003; Kerns et al. 2004). There is also evidence that differences in vulnerability are a factor in the impact of stress on sleep, but the significance of individual differences has not been fully appreciated in either studies of stress or in studies of sleep in general (Irmis et al. 1971, 1974; Tang et al. 2007).
Some of the best evidence for the role in resilience and vulnerability in the impact of stress on sleep comes from studies comparing inbred strains of rodents, which are genetically identical within strain but which vary genetically and phenotypically across strain. Work in mice and rats has demonstrated that strains that exhibited greater anxiety-like behaviors in response to challenges in wakefulness exhibited correspondingly greater and longer duration alterations in sleep after training with IS and after fearful cues (Sanford et al. 2003c) and contexts (Sanford et al. 2003a) associated with IS. In general, vulnerable mouse strains (e.g., BALB/cJ mice compared to more resilient C57BL/6 J mice) also showed greater decreases in sleep after stressful situations with unlearned responses, including exposure to an open field (Tang et al. 2004), cage change, and novel objects placed in the home cage (Tang et al. 2005b). Moreover, BALB/cJ mice also do not show a significant REM sleep increase during recovery from restraint stress, whereas C57BL/6 J mice do (Meerlo et al. 2001b).
In addition to genetic determinants of individual resilience and vulnerability, environmental factors and prior experiences with stress also play a major role in shaping future responses to stressful challenges. One such factor is neonatal stress that can be induced by disruptions of mother–infant relationship and that can have repercussions for adult behavior (Levine 2005) though the specific effects of maternal separation on sleep have varied across studies. For example, 3 h of maternal separation from postnatal days 2–14 in rats has been reported to increase both spontaneous baseline REM sleep and cold-stress-induced changes in REM sleep in males (Tiba et al. 2004) and females (Tiba et al. 2008). Similarly, neonatal rats maternally separated for 3 h and exposed to chronic mild stress as adults were reported to show longer sleep time, more episodes of REM sleep, and more episodes of NREM sleep transitioning to REM sleep (Mrdalj et al. 2013). By comparison, 6 h of neonatal maternal deprivation reduced the time spent in REM, without changes in NREM sleep when the rats attained adulthood (Feng et al. 2012). In addition to differences in experimental procedure, another important aspect that may differentiate these studies is the strain of rats used. While Feng and co-workers used Sprague-Dawley rats, the other studies used Wistar rats, which display more maternal behavior upon reunion with their litters (Lehmann and Feldon 2000), possibly buffering potential harmful effects of the separation procedure.
4 Stress Mediators as an Important Cause of Arousal and Sleep Disturbance
The regulation of sleep and arousal involves multiple neurotransmitter systems as well as excitatory and inhibitory amino acids, peptides, purines, and neuronal and non-neuronal humoral (i.e., cytokines and prostaglandins) modulators (Steiger and Holsboer 1997; Steiger et al. 1998; Jones 2005; Steiger 2007; Luppi 2010; Espana and Scammell 2011). Many of these same neurotransmitters and neuromodulators are also influenced by and/or mediate the effects of stress and are likely involved in the effects of stress on sleep. This section will briefly review some of the major neurochemical systems that link stress and sleep.
4.1 Hypocretin/Orexin
Hypocretin-1 and -2 are a set of neuropeptides that are derived from the same precursor gene and produced by neurons located in the lateral hypothalamus. The hypocretins are also called orexins as they were independently discovered by two research groups in 1998 and separately named as hypocretins (de Lecea et al. 1998) or orexins (Sakurai et al. 1998). The hypocretin containing neurons have widespread projections throughout the brain and play a role in a variety of functions including autonomic control, neuroendocrine function, and feeding. Numerous studies have also linked hypocretin to the regulation of the sleep–wake cycle, particularly the induction and maintenance of wakefulness (Kilduff and Peyron 2000; Sutcliffe and de Lecea 2002). Indeed, the hypocretin system activates various well-known wake-active and arousal promoting centers in the brain, including the histaminergic tuberomammilary nucleus, the noradrenergic locus coeruleus (LC), the serotonergic dorsal raphe, and the cholinergic cell clusters in the brainstem and basal forebrain (Peyron et al. 1998b). Impaired hypocretin transmission is a core pathophysiological factor of narcolepsy, a disease characterized by uncontrollable onset of sleep (Nishino et al. 2000; Kornum et al. 2011).
Several lines of evidence indicate that hypocretins/orexins may also play a role in the behavioral arousal and neuroendocrine activation associated with stress (Winsky-Sommerer et al. 2005). A close and bidirectional relationship exists between the hypocretin system and the HPA axis. Hypocretins stimulate the activity of the HPA axis in a dose-dependent manner, an effect that seems to be mediated at the hypothalamic level (Kuru et al. 2000; Samson et al. 2002) but not at the adrenal level (Jaszberenyi et al. 2000). Under stressful conditions, a dual hypocretin-1/hypocretin-2 receptor antagonist does not interfere with corticosterone secretion but does reverse the immediate waking effect of novelty and social stressors (Steiner et al. 2013). In turn, hypothalamic corticotropin releasing hormone (CRH) containing neurons project directly to the lateral hypothalamus hypocretin containing neurons, where CRH1 and 2 receptors are abundantly expressed (Winsky-Sommerer et al. 2004). Indeed, studies in mice have shown that exposure to footshock and restraint stress causes an activation of the lateral hypothalamic hypocretin neurons, an effect that is mediated by CRH (Winsky-Sommerer et al. 2005).
4.2 Corticotropin Releasing Hormone
CRH is a major mediator of central nervous system responses to stressors (Koob and Bloom 1985; Heinrichs et al. 1995; Koob 1999). Intracerebroventricular (ICV) administration of CRH in rats produces many of the signs associated with anxiety in humans, including increased wakefulness (Ehlers et al. 1986; Marrosu et al. 1990; Chang and Opp 1998), altered locomotor activity, and an exaggerated startle response (Swerdlow et al. 1986; Heilig et al. 1994). By comparison, CRH antagonists attenuate behavioral responses to stress (e.g. Aloisi et al. 1999; Basso et al. 1999; Deak et al. 1999).
CRH may not only play an important role in stress-induced wakefulness and arousal, it may also be partly responsible for changes in sleep architecture during the subsequent recovery phase (Gonzalez and Valatx 1997). However, the few studies examining the role of CRH in stress-induced alterations in sleep have yielded conflicting data. This is exemplified with the work on restraint stress-induced increases in REM sleep. The ICV administration of the broad CRH antagonist α-helical CRH9–41 prior to restraint stress prevents the subsequent increase in REM, but does not alter spontaneous REM, NREM, or wakefulness in non-stressed rats (Gonzalez and Valatx 1997). In contrast, other investigators found no effect of restraint stress applied at the beginning of the dark period on subsequent sleep, and also found no effect of the CRH antagonist, astressin, on sleep after restraint (Chang and Opp 2002). By comparison, restraint exposure at the onset of the light period increases wakefulness and decreases both NREM and REM, and ICV administration of astressin attenuates the increase in wakefulness over a 5 h-period immediately after the end of restraint but does not alter arousal during the period when restraint was applied (Chang and Opp 2002). There may have been differences in the procedures used for restraint [e.g., whether or not it was conducted in the home cage (Chang and Opp 2002)] that could have produced different results in these studies.
A recent study (Kimura et al. 2010) examined baseline and recovery sleep after sleep deprivation in conditional mouse mutants that overexpress CRH in the entire central nervous system or only in the forebrain, including limbic structures. In baseline recordings, homozygous mice with either global or forebrain overexpression of CRH showed increased REM compared to controls and both homozygous and heterozygous mice with global overexpression of CRH showed enhanced recovery REM sleep after 6 h sleep deprivation. However, repeated ICV administration of CRH during prolonged REM sleep deprivation in rats inhibits the expected REM rebound (Machado et al. 2010). Enhanced REM sleep recovery, but not NREM sleep recovery, was blocked by oral administration of the CRH receptor type 1 (CRHR1) antagonist, DMP696, 1 h prior to the end of sleep deprivation. Peripheral stress hormone levels were not elevated during baseline and did not differ across genotypes after sleep deprivation. The authors concluded that enhanced REM sleep in these mice was most likely induced through the activation of CRHR1. Consistent with this conclusion is a report that repeated administration of α-helical CRH9–41 in rats over 10 h of sleep deprivation also reduced the amount of REM sleep recovery (Gonzalez and Valatx 1998).
However, there is a separate line of research that demonstrates an inhibiting effect of CRH on REM sleep. Fear conditioning with IS, an uncontrollable stressor, and the presentation of fearful contexts and cues associated with IS are followed by significant reductions in REM that occur in the first few hours after exposure (Sanford et al. 2003a, c). In mice, ICV administration of CRH enhances the reduction in REM sleep following fearful contexts, whereas ICV administration of the non specific CRH antagonist, astressin, attenuates fear-induced reductions in REM (Yang et al. 2009). Training with ES, and reminders of ES, can produce significant enhancements in REM sleep (Sanford et al. 2010). Microinjections of either saline or astressin prior to training produce similar, significant enhancements in post-stress REM sleep relative to a non-shocked handling control condition, whereas the increases in REM sleep are blocked by pretreatment with CRH (Yang et al. 2011b). The effect of CRH seems to be relatively specific for REM sleep as changes in NREM sleep and wakefulness were minimal. One potential explanation for differences across studies is that administration of a CRH antagonist simply blocked the initiation of neural processes that would have led to a subsequent increase in REM sleep.
4.3 Prolactin
A variety of studies have indicated that prolactin can promote REM sleep. Both systemic and ICV injection of prolactin enhances REM sleep in rats (Roky et al. 1995), whereas administration of a prolactin antiserum reduces the amount REM sleep without affecting NREM sleep (Obal et al. 1992). Also, the amount of REM sleep was found to be reduced in prolactin-deficient mice, which could be reversed by prolactin replacement (Obal et al. 2005).
Several studies in laboratory rodents have shown that the plasma level of prolactin increases in response to a wide variety of stressors, including restraint stress and ether exposure (Lenox et al. 1980; Meerlo et al. 2001b) suggesting that it may play a role in the effects of stress on sleep. A comparative study on different strains of mice showed that C57BL/6 J mice and BALB/cJ mice had similar corticosterone responses to restraint stress; however, the effects on prolactin and subsequent sleep were quite different. Restraint stress caused a concomitant increase in prolactin and REM sleep in the C57BL/6 J mice, but not in BALB/cJ mice, which supports the idea that prolactin might be involved in the mechanism underlying restraint stress-induced REM sleep (Meerlo et al. 2001b). Direct evidence for prolactin as a mediator of stress-related increases in REM sleep comes from a study in rats showing that an ether exposure-induced increase in REM sleep could be blocked by hypophysectomy and by ICV administration of an antiserum to prolactin (Bodosi et al. 2000). Other data implicating prolactin in stress-induced alterations in sleep come from a study examining post-stress sleep in REM sleep-deprived rats subsequently submitted to single or repeated sessions of footshock (Machado et al. 2008). REM sleep rebound was greater in the REM sleep-deprived rats that received multiple sessions of footshock, and the increase was associated with higher levels of prolactin (Machado et al. 2008).
Together these studies suggest that stressful stimuli and conditions that are associated with strong increases in prolactin levels may be followed by sleep with increased amounts of REM sleep. The precise mechanism of these effects of prolactin remains to be clarified but may involve a direct stimulatory effect of prolactin on cholinergic neurons in the mesopontine tegmental area involved in REM-sleep induction (Takahashi et al. 2000).
4.4 Monoamines
Serotonin (5-HT) containing neurons in the dorsal raphe nucleus (DRN), noradrenaline (NA) containing neurons in the LC, and histamine containing neurons in the tuberomammillary nucleus are wake-active and act directly on cortical and subcortical regions to promote wakefulness (Jones 2005). The 5-HT and NA systems are strongly stress-reactive (see discussion below) whereas there has been less work on the role of the histaminergic system. However, it is involved with the regulation of the stress response as central administration of histamine produces increases in adrenocorticotropin and corticosterone (Rudolph et al. 1979; Knigge and Warberg 1991) and blocking histamine synthesis or administration of antagonists block ACTH, beta-endorphin and prolactin responses to some stressors (Rudolph et al. 1979; Seltzer et al. 1986; Knigge and Warberg 1991; Kjaer et al. 1993; Fleckenstein et al. 1994). There also appears to be heterogeneity in specific histaminergic cells groups with respect to responding to different stressors (Miklos and Kovacs 2003).
Interestingly, each of these systems has been implicated in the enhancement of REM sleep that typically follows restraint stress. The increase is not found in 5-HT1A knockout mice (Boutrel et al. 2002; Popa et al. 2006) or in mice lacking the 5-HT transporter (Rachalski et al. 2009). Administration of the serotonin synthesis inhibitor para-chlorophenylalanine (Sinha 2006), neurotoxic destruction of noradrenergic cells in LC (Gonzalez et al. 1995), and administration of the histamine H1 receptor antagonist, chlorpheniramine, also prevent the increase in REM sleep induced by restraint in rats (Rojas-Zamorano et al. 2009). However, the actual cause of the attenuation of the REM sleep increase is not yet fully understood. As indicated for CRH, alterations in these systems prior to stress could simply alter the intensity of some elements of the stress response such that the processes that result in the post-restraint increase in REM sleep are not engaged.
5 Brain Regions Linking Stress, Arousal and Sleep
As discussed in the above section on stress mediators, there are several points of overlap in the neural regions/systems involved in stress and those directly involved in arousal. This section will focus on the amygdala and medial prefrontal cortex (mPFC), two regions not typically considered as direct regulators of arousal and sleep but which play significant roles in mediating the effects of stress on sleep and arousal (see Fig. 2).
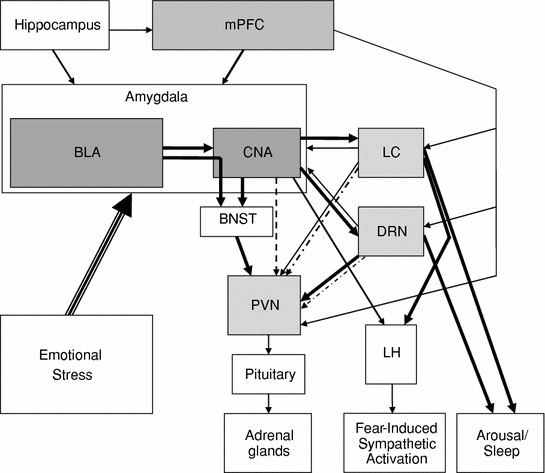
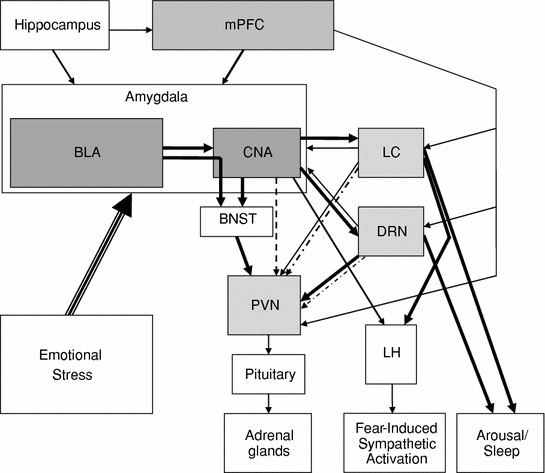
Fig. 2
This diagram illustrates the principal circuitry (shaded) that we are discussing in this section along with some of their connections to other regions involved in stress and sleep. In this figure, emotional stress would act on the amygdala which would be regulated by the hippocampus (contextual information) and the mPFC (perceived stressor control). BLA would act on CNA and the BNST to regulate the peripheral stress axis via PVN. Output from CNA would also impact LC and DRN, which have roles in regulating REM sleep and arousal as well as in regulating PVN. Both CNA and LC are involved in regulating fear-induced sympathetic activation via effects on LH. This diagram is necessarily incomplete, but illustrates the central role of the amygdala in controlling the stress axis, fear responses, and important components of the arousal system. Heavyweight arrows indicate presumed critical connections for mediating the effects of stress on sleep. Lightweight arrows indicate other connections that may play a role in regulating responses. Dashed arrows indicate indirect pathways. BLA basolateral nucleus of the amygdala, BNST bed nucleus of the stria terminalis, CNA central nucleus of the amygdala, DRN dorsal raphe nucleus, LC locus coeruleus, LH lateral hypothalamus, mPFC medial prefrontal cortex, PVN paraventricular nucleus
5.1 Amygdala and Stress-Induced Alterations in Arousal and Sleep
Several lines of research have demonstrated that the amygdala is a significant modulator of sleep. The majority of research on the role of the amygdala in regulating sleep has focused on its influence on REM sleep (e.g. Sanford et al. 1995, 1998, 2002; Calvo et al. 1996; Zhu et al. 1998; ); however, a number of studies indicate that the amygdala can influence all sleep–wakefulness states (Sanford et al. 1995; 1998, 2006; Zhu et al. 1998). This influence most likely involves amygdalar projections to thalamic, hypothalamic, and brainstem target regions (Amaral et al. 1992) that are involved in the control of sleep and arousal. These include direct projections via the central nucleus of the amygdala [CNA; e.g. (Krettek and Price 1978; Inagaki et al. 1983; Price et al. 1987; Semba and Fibiger 1992; Peyron et al. 1998a)] and the lateral division of the bed nucleus of the stria terminalis [BNST; reviewed in (Amaral et al. 1992; Davis and Whalen 2001)], the source of the major descending outputs of the amygdala to brainstem regions linked to the regulation of REM sleep.
The amygdala is important in the regulation of behavioral, physiological, and neuroendocrine responses to stress (Roozendaal et al. 1991a, b; Bohus et al. 1996) and it appears to be a vital interface between stressful events and their impact on sleep and arousal. The BNST is an important relay for the influence of the amygdala on the hypothalamic paraventricular nucleus (PVN) (Forray and Gysling 2004), the final common pathway for information influencing the HPA axis (Pacak and Palkovits 2001; Herman et al. 2004) and a key site for integrating neuroendocrine, autonomic, and behavioral responses to stress (Chrousos 1998). GABA-ergic neurons in BNST can directly inhibit PVN and reduce ACTH secretion (Herman et al. 2004). By comparison, CNA has minimal direct projections to PVN (Prewitt and Herman 1998) and lesions of CNA do not directly influence PVN activation (Prewitt and Herman 1997) though CNA can influence PVN via trans-synaptic pathways through the dorsomedial hypothalamic nucleus and BNST (Prewitt and Herman 1998).
CNA does play a role in regulating the effects of stress on sleep, whereas a possible role for BNST has not been established. Inhibition of the CNA suppresses REM sleep whereas its activation [e.g., with electrical stimulation (Smith and Miskiman 1975)] can promote REM sleep in some situations. For example, functional inactivation of CNA with microinjections of the GABAA agonist, muscimol, produces a relatively selective decrease in REM sleep whereas blocking GABAergic inhibition with the GABAA antagonist, bicuculline, enhances REM sleep (Sanford et al. 2002). Functional lesions of the CNA by TTX, which inactivates both cell bodies and fibers of passage also decrease REM sleep and reduce arousal (Tang et al. 2005c). The decrease in REM sleep can occur without recovery (Tang et al. 2005c), a finding also reported for training with IS and fearful cues and contexts.
That stress-induced inactivation of CNA is involved in stress-induced reductions in REM sleep is also suggested by the lack of Fos activation in CNA after conditioned fear (Liu et al. 2003). Functionally, this hypothesis is supported by findings that bicuculline microinjected into CNA attenuates footshock-induced reductions in REM sleep whereas inactivation of CNA with muscimol did not (Liu et al. 2009). However, it should be noted that findings that activation of CNA promotes and inactivation of CNA reduces REM sleep appear at odds with the prevailing conventional view that CNA activation is responsible for regulating fear responses via projections to the periaqueductal gray and other brainstem areas [Reviewed in (Duvarci et al. 2011)]. In fact, CNA neurons do fire in response to footshock stress (Rosenkranz et al. 2006) and in response to conditioned stimuli (Duvarci et al. 2011). However, CNA is inhibited by stimulation of the basal and lateral nuclei of the amygdala (Rosenkranz et al. 2006) both of which show high Fos expression after footshock (Liu et al. 2003). Thus, it is possible that CNA activation during fearful/stressful events does regulate fear behavior in wakefulness, but subsequently, with certain stressors, can be inhibited to decrease REM sleep in the post-stress period.
The involvement of the basolateral nucleus of the amygdala (BLA) in the control of sleep is indicated by reports that bilateral electrolytic and chemical lesions of BLA increase NREM sleep and total sleep time in rats (Zhu et al. 1998) and that bilateral chemical lesions of the amygdala produce more consolidated sleep in chair restrained Rhesus monkeys (Benca et al. 2000). Electrical and chemical stimulation of BLA also increase low voltage, high frequency activity in the cortical EEG and decrease NREM sleep and total sleep time, respectively (Dringenberg and Vanderwolf 1996; Zhu et al. 1998).
In general, the evidence suggests that CNA is more involved in the regulation of REM sleep than that of NREM sleep and that by comparison, BLA has a greater role in the regulation of NREM sleep and arousal. However, it is important to note that BLA regulates CNA output and therefore likely controls its influences on REM. Fibers from BLA also course through CNA on to the BNST which has brainstem targets similar to those of CNA (Davis and Whalen 2001), thereby providing an additional pathway by which BLA can influence brainstem regions. Indeed, it was recently found that microinjections into BLA of the Group II metabotropic glutamate (mGlu) receptor agonist, LY379268, selectively reduced REM sleep without significantly altering wakefulness or NREM sleep (Dong et al. 2012). By comparison, microinjection of LY379268 into CNA did not significantly alter sleep. Thus, group II mGlu receptors may influence specific cells in BLA that control descending outputs (possibly via CNA or BNST) that in turn regulate REM sleep generator regions in the brainstem.
The amygdala (including extended amygdala) is a critical region for the central effects of CRH, and it appears to mediate a number of the anxiogenic effects of CRH as evidenced by intra-amygdala microinjections of CRH agonists and antagonists [Reviewed in (Davis and Whalen 2001)]. For example, local application of CRH or urocortin (Sajdyk et al. 1999) into the BLA in rats produces dose-dependent increases in anxiety behaviors. CRH in the amygdala also plays a significant role in regulating stress-induced alterations in sleep.
It was reported that microinjections of the CRHR1 antagonist, antalarmin, into CNA in rats block fear-induced reductions in REM sleep and attenuate Fos expression in regions important in stress and REM sleep regulation including the PVN, LC, and DRN (Liu et al. 2011). Similarly, bilateral microinjections of antalarmin into BLA in rats do not alter spontaneous sleep, but do block the reduction in REM sleep produced by inescapable footshock (Wellman et al. 2013). Further, microinjecting antalarmin into BLA prior to shock training also blocked the subsequent effects of contextual fear on REM sleep, but did not block fear memory or behavior as indicated by freezing. These data indicate that CRH receptors within the CNA and BLA are important in the regulation of stress- and fear-induced alterations in REM sleep, and also suggest that BLA plays a role in modulating how stressful memories influence sleep. The hippocampus is also likely to be involved. Information regarding fear conditioned contexts is first processed in the hippocampus and followed by BLA with output through CNA [reviewed in (LeDoux 2000)] and possibly BNST (Davis and Whalen 2001).
5.2 REM Regulatory Regions, Medial Prefrontal Cortex and Stressor Control
Stress often has a prominent effect on REM sleep (Sanford et al. 2003a, b, c; Jha et al. 2005). Thus, it is not surprising that brain regions directly implicated in the regulation REM sleep have significant roles in mediating the stress response. These include the LC and DRN. LC noradrenergic neurons and DRN serotonergic neurons are virtually silent during REM sleep and their activation may inhibit its generation (Steriade and McCarley 1990). LC and DRN also have connections to the PVN. PVN receives a large noradrenergic projection from brainstem A1 and A2 groups and a smaller projection from LC (Dunn et al. 2004). However, lesions of LC do reduce ACTH and corticosterone responses to acute stress (Ziegler et al. 1999), and there are suggestions that LC may impact PVN indirectly via limbic structures [reviewed in (Herman and Cullinan 1997)]. DRN has collateral serotonergic projections to CNA and PVN (Petrov et al. 1992, 1994), and 5-HT agonists enhance PVN activity as indicated by increased corticosterone levels and Fos expression (Mikkelsen et al. 2004). Indirect pathways may also play a role in serotonergic regulation of PVN (Herman and Cullinan 1997).
As discussed above, stressor controllability appears to be an important parameter in the effects of stress on sleep. Brainstem noradrenergic and serotonergic regions play important roles in stressor controllability. For example, IS in rats produced sustained increases in NA turnover in various brain regions regardless of stress duration, whereas with ES, NA utilization was reduced after rats had learned the coping response (Tsuda et al. 1987). IS also activates DRN serotonergic neurons to a greater degree than ES thereby increasing 5-HT in DRN and in target areas (Amat et al. 1998a, b; Hammack et al. 2002; Bland et al. 2003a, b). Administration of CRH into DRN produced behavioral changes like those seen with IS, whereas microinjection of a nonselective CRH antagonist blocked the behavioral changes normally seen with IS (Hammack et al. 2002, 2003a, b). The application of CRH to LC also increases NA release (Van Bockstaele et al. 1998).
The alterations in 5-HT and NA after inescapable and ES are consistent with their putative roles as inhibitory modulators of REM sleep and with the respective increases and decreases in REM sleep after controllable and uncontrollable stress. However, the evaluation of controllability requires assessment and evaluation of information at the cortical level (Maier et al. 2006). The mPFC has been found to be a critical region in the perception of control and in mediating the consequences of stress (Maier et al. 2006; Smith and Vale 2006; Akirav and Maroun 2007). For example, blocking activation of the ventral mPFC with muscimol did not alter escape behavior in rats presented with IS, but blocking ventral mPFC in rats presented with ES produced failure in escape learning and greater fear conditioning (Maier et al. 2006). By comparison, activation of ventral mPFC with picrotoxin prior to IS promoted later escape learning in rats provided an opportunity to escape shock in a shuttlebox (Maier et al. 2006).
Unfortunately, the role of the mPFC in mediating the effects of stressor controllability on sleep has not been examined. However, part of the influence of mPFC is enacted through its effects on the DRN and possibly LC (Maier et al. 2006), providing a potential substrate for regulating alterations in REM sleep. For example, consistent with the discussion above, activation of mPFC inhibits DRN (Maier et al. 2006; Smith and Vale 2006). The prelimbic mPFC also has robust but restricted projections to the BLA and CNA, whereas the infralimbic mPFC projects to the medial, basomedial, cortical nuclei as well as to the CNA (Vertes 2004). There are projections from the mPFC to GABAergic neurons in the intercalated nuclei which have inhibitory control over CNA output, but there are conflicting reports regarding their specific origin within mPFC (see Vertes 2004). However, these projections from the mPFC to brainstem regulatory regions and the amygdala provide a substrate by which stressor controllability could influence REM sleep.
6 Stress, Sleep and Neuronal Plasticity: Implications for Stress-Related Disorders
Both NREM and REM sleep have putative roles in regulating neuronal plasticity and synaptic strength (Benington and Frank 2003; Tononi and Cirelli 2006; Meerlo et al. 2009; Havekes et al. 2012). Stress-induced changes in sleep and sleep architecture might lead to alterations in these plasticity processes and ultimately brain function. In fact, some of the changes in plasticity and brain function traditionally linked to stress may in part be related to alterations in sleep.
Work on stress and plasticity has distinguished the effects of acute and chronic stress. Acute stress can impact functional plasticity whereas chronic stress can differentially alter structural plasticity across brain regions. For example, chronic stress results in dendritic atrophy and reductions in spine density in the hippocampus (Magarinos and McEwen 1995a, b; Magarinos et al. 1997; Sandi et al. 2003; Stewart et al. 2005) and prefrontal cortex (Wellman 2001; Cook and Wellman 2004; Radley and Morrison 2005; Liston et al. 2006; Radley et al. 2006). Similar types of chronic stress produce increased dendritic arborization and increased spine density in BLA spiny neurons (Mitra et al. 2005; Vyas et al. 2006) and spine down-regulation in the medial amygdala (Bennur et al. 2007). Some stress-induced changes in the hippocampus (Sandi et al. 2003; Stewart et al. 2005) and prefrontal cortex appear to be reversible whereas those in the amygdala are not [Reviewed in (Christoffel et al. 2011)]. Acute restraint, tail shock, and environmental stress impair long-term potentiation (LTP) in the hippocampus (Foy et al. 1987) and acute environmental stress can enhance long-term depression (Xu et al. 1997). However, stress-induced impairment in hippocampal LTP was significantly less in rats allowed to escape footshock than in yoked controls receiving identical shock, but not allowed to escape (Shors et al. 1989). This control mediated attenuation occurred even after a week of daily training sessions with relatively intense shock (30 trials, 1 mA, 1.5 s mean duration).
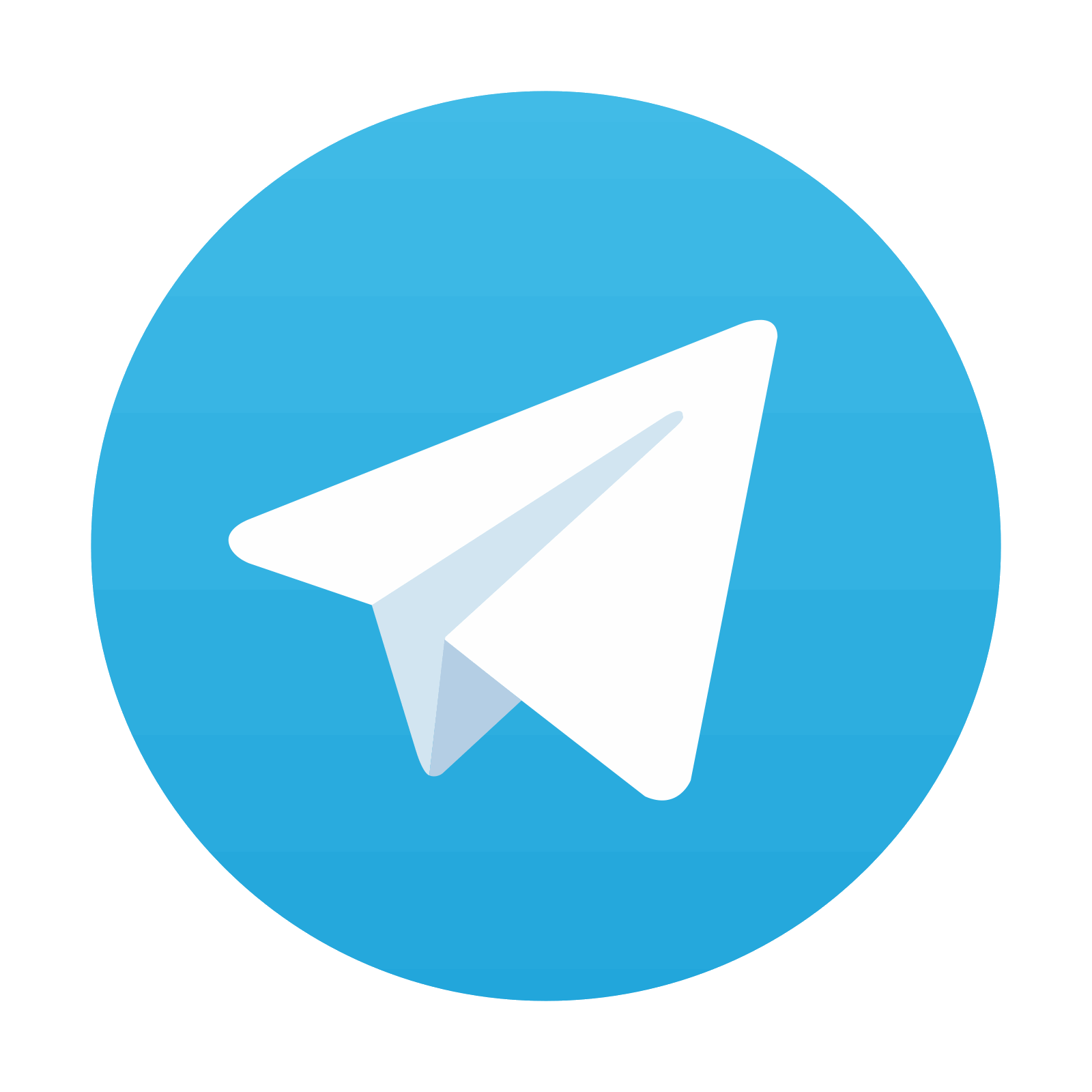
Stay updated, free articles. Join our Telegram channel
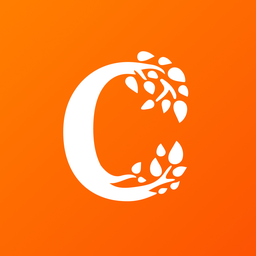
Full access? Get Clinical Tree
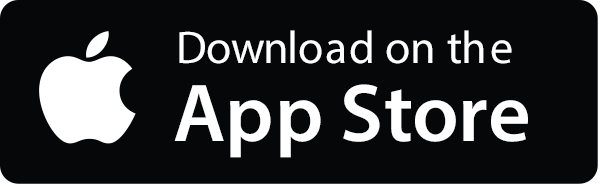
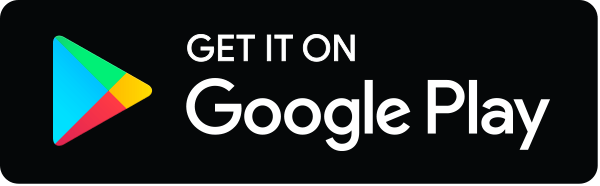