Fig. 18.1
Metabolic pathway of adenosine. See text for details; NT, nucleoside transporter
Both neurons and glia can influence extracellular adenosine concentration. Direct evidence demonstrating that astrocytes are a source of extracellular adenosine in the brain through ATP release comes from the work of Haydon and colleagues. They generated an inducible transgenic mouse line, termed the dnSNARE mouse, with astrocyte-specific expression of a dominant-negative domain of the soluble N-ethylmaleimide-sensitive factor attachment protein receptor (SNARE) (Pascual et al. 2005) . This mouse has disrupted vesicular release of gliotransmitters, and extracellular ATP and adenosine concentration in brain slices of these mice is found to be significantly reduced (Pascual et al. 2005; Schmitt et al. 2012) . Consequently, dnSNARE mice exhibit attenuated adenosine-mediated presynaptic inhibition, which can be reconstituted by application of exogenous ATP (Pascual et al. 2005) . These results support the idea that astroglial ATP vesicular release is a major source of extracellular adenosine. In addition, astrocytes can also contribute to extracellular adenosine through ATP release via Cx hemichannels and pannexin channels, especially in pathological conditions (Kang et al. 2008; Garré et al. 2010; Iglesias et al. 2009; Orellana et al. 2011) . Given also the fact that AK is almost exclusively expressed in astrocytes in the adult mouse brain (Studer et al. 2006) , these glial cells are likely to be a key regulator of extracellular adenosine levels by an adenosine cycle involving release of ATP, uptake of extracellular adenosine degraded from ATP via NTs and conversion of intracellular adenosine to AMP by AK (Boison 2008) .
18.3.3 Astrocytes and Sleep Homeostatic Regulation
Considering their importance in regulating adenosine levels, it is logical to speculate that astrocytes are involved in adenosine-dependent sleep regulation. Indeed, EEG/EMG recordings revealed that the dnSNARE mouse has reduced SWA during basal NREM sleep and significantly attenuated increase in SWA and recovery sleep after SD (Halassa et al. 2009) . Intracerebroventricular infusion of an A1R antagonist produced similar phenotype in wild type mice suggesting that astrocytic adenosine acts on A1R to modulate sleep pressure (Halassa et al. 2009). In agreement with this view, both conditional knockout of A1R (Bjorness et al. 2009) and overexpression of AK that reduces extracellular adenosine (Palchykova et al. 2010) cause sleep homeostasis phenotypes similar to those of the dnSNARE mice.
There are several lines of evidence suggesting that adenosine might be involved in some sleep disorders . For example, adenosine increase during SD and subsequent sleep recovery is attenuated in old rats (Wigren et al. 2009) , and binding of adenosine receptor decreases in aged humans, possibly due to loss of A1R (Meyer et al. 2007) . Also, it is found that rats exposed to sleep fragmentation, a likely cause of excessive daytime sleepiness in patients of sleep apnea and other sleep disorders, exhibit elevated sleepiness and show rise of adenosine in the basal forebrain (McKenna et al. 2007) . Studies of humans have shown that a single polymorphism of the adenosine deaminase gene leads to lower enzymatic activity of adenosine deaminase and presumably higher adenosine levels. Subjects with this polymorphism have enhanced slow wave sleep and sleep pressure (Bachmann et al. 2012) . Finally, Hines and colleagues, using the dnSNARE mouse showed that astrocyte signaling to A1R is required for 12 h SD-induced alleviation of depressive-like behaviors in mouse model of major depression, and that the effect can be mimicked by administration of an A1R agonist (Hines et al. 2013). Considering the high co-occurrence of sleep disorders and depression (Nutt et al. 2008) , their study implicates that astrocyte-derived adenosine might be involved in sleep disorders.
Taken together, malfunction of astroglial adenosine signaling and metabolism could be as yet unidentified causes of certain primary sleep disorders or secondary sleep problems associated with other diseases. Thus, the cellular machinery of the astrocyte signaling and metabolic pathways could represent potential therapeutic targets that should be worthy of research attention.
18.4 Neuroglial Metabolic Coupling and Sleep
18.4.1 Sleep and Metabolism
Sustenance of brain functions is energy expensive; 20 % of the total energy produced by the body is consumed by the brain, which represents only 2 % of the body mass (Magistretti 2008). One of hypothetic functions of sleep is to restore energy spent during wakefulness (Frank 2010) . In agreement with this view, overall metabolic rate of the cerebral cortex is reduced during NREM sleep (Maquet 1995) . Interestingly, the prime energy source of the brain, i.e. extracellular glucose, increases during NREM sleep and decreases during wakefulness and REM sleep (Netchiporouk et al. 2001; Dash et al. 2013) . Moreover, sleep impairments are associated with higher risks of pathological metabolism, such as obesity , diabetes , hypertension, etc. (Porkka-Heiskanen et al. 2003; Spiegel et al. 2009) . Apart from peripheral conditions, functional imaging also revealed a variety of brain areas that undergo abnormal metabolic states in patients of major sleep disorders including insomnia and catalepsy (Desseilles et al. 2008) .
18.4.2 Astrocytes and Brain Metabolism
The level of neural activity is tightly coupled with brain metabolic rate. Both glucose utilization and blood flow increase with neural activity, i.e. during cognitive tasks . For the past decades, intense research efforts have demonstrated that astrocytes play a central role in neuro-vascular metabolic coupling. Astrocytes are structurally adapted and perfectly positioned to support synaptic activity, which constitutes the largest brain energy expenditure (Bélanger et al. 2011). Indeed, astrocytes extend perisynaptic processes closely contacting synapses (Ventura and Harris 1999) which contain neurotransmitter receptors and transporters to sense synaptic activity. On the other hand, astroglial vascular processes, called endfeet, enwrap blood vessels and express glucose transporters to control blood flow and uptake of glucose (Allaman et al. 2011) . How astrocytes respond to neuronal activity and supply energy needs of neurons is theorized by the astrocyte-neuron lactate shuttle hypothesis (Pellerin and Magistretti 1994) . Briefly, synaptic activity increases extracellular glutamate, which is taken up by astrocyte plasmalemmal glutamate transporters, and results in an increase in [Na+]i; which stimulates Na+/K+ pump and increases astroglial consumption of ATP . This in turn triggers astroglial glucose uptake from circulation and glycolysis, which produces lactate; the latter being released and taken up by neurons as the energy substrate. This model has been supported by numerous in vitro and in vivo studies (Barros et al. 2009; Cholet at al. 2001; Voutsinos-Porche et al. 2003; Chuquet at al. 2010; Lin et al. 2010; Rouach et al. 2008) , indicating that neuronal activation is accompanied by sustained production of lactate by astrocytes and that lactate, instead of glucose, is the preferred energy substrate of neurons .
18.4.3 Astrocyte-Derived Lactate and Sleep
It has been observed that cortical lactate concentration rises upon waking up and maintains elevated during continuous wakefulness and that it persistently declines during NREM sleep (Naylor et al. 2012). Also, cerebral SWA decreases the rate of glycolysis, which produces lactate (Wisor et al. 2012) . Moreover, the NREM-associated decline of lactate reflects sleep pressure (Dash et al. 2012) . Lactate levels are not only correlated with sleep-wake changes, but there is also evidence that lactate affects excitability of the orexin (wakefulness-promoting) neurons in the lateral hypothalamic nuclei. These neurons fire during wakening and are silent during sleep, moreover, loss of orexin neurons or orexin receptors is an established cause of narcolepsy (Lin et al. 1999; Chemelli et al. 1999) . Orexin neurons are essentially astroglial lactate sensors. They specifically utilize lactate released by astrocytes, their spontaneous firing rate is correlated with lactate concentration and lactate increases their sensitivity to excitatory inputs (Parsons and Hirasawa 2010) . The effects of lactate on orexin neuron behavior, thus, indicate a possible mechanism by which astrocyte-derived lactate could influence sleep regulation.
So far, we have only focused on lactate, which is only one of the possible links between neuroglial metabolic coupling and sleep. There are many other players of astroglial metabolism, including glucose, ATP, etc., that may also influence this process. Considering the vital roles of astrocytes in brain metabolism, it is tantalizing to hypothesize that astrocytes may be indirectly involved in sleep physiology and pathology via their metabolic functions, although this remains largely unclear and needs investigation.
18.5 Astroglial Connexins and Sleep
18.5.1 Connexins, Hemichannels, Gap Junction Channels and Communicating Networks of Astrocytes
Astrocytes are organized in networks of cells connected by gap junction channels (Fig. 18.2). These astroglial networks are involved in the homeostasis of ions, glutamate and second messengers and in the removal of toxic substances (Giaume et al. 2010) . They contribute to the intercellular trafficking of energy metabolites to neurons and sustain synaptic activity (Rouach et al. 2008) . In the brain, astrocytes are the cell population that exhibits the highest level of Cx expression. Although a minor expression of a third Cx, Cx26, cannot be excluded in sub-populations of astrocytes (Mercier and Hatton 2001; Nagy et al. 2001, 2011; but see Filippov et al. 2003) , the two prevalent astroglial Cxs are Cx30 and Cx43. This has been confirmed using mice double knock out of Cx30 and Cx43 in which gap junctional communication assessed by dye coupling in hippocampal astrocytes is totally abolished (Wallraff et al. 2006; Rouach et al. 2008) .
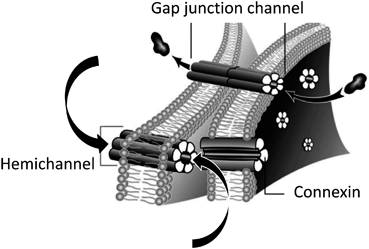
Fig. 18.2
Connexin-based gap junction channels and hemichannels. The upper arrows indicate the direct intercellular exchanges of ions, low molecular weight signaling molecules and metabolites through a gap junction. The two lower arrows indicate that the permeants though hemichannels can be either taken up or released by astrocytes
Connexins aggregate at junctional plaques and form hexameric rings of Cxs, termed connexons or hemichannels. Hemichannels were initially assumed to remain closed (Fig. 18.2), however, functional Cx hemichannels were later demonstrated to exist in astrocytes enabling astrocytes to play new roles in neuroglial interaction (Bennett et al. 2003; Giaume et al. 2013) . Connexin hemichannels are permeable to glucose and represent an alternative pathway for the uptake and the release of energy metabolites (Retamal et al. 2007) .They are also permeable to neurotransmitters such as glutamate (Yé et al. 2003) and ATP (Kang et al. 2008) , thus playing a role in autocrine and paracrine brain communication. In diverse pathological situations, the pattern of Cx expression, the extent of gap junctional communication and the hemichannel activity are modified in astrocytes, as recently reviewed (Giaume et al. 2010, 2013) . These changes depend on the type and severity of insult, the distance from the lesion site and the time post-injury. As a consequence, both deleterious and protective effects have been reported, in particular after ischemia , stroke or trauma (Giaume et al. 2010) .
18.5.2 Astroglial Connexins Could Be Involved in Sleep-Wake Regulation
Several findings support that possible changes in Cx channel-mediated communication could be related to sleep regulation. General anesthetics (Mantz et al. 1993). Oleamide, a sleep-inducing lipid (Guan et al. 1997) , have been reported to inhibit gap junctions in primary cultures of astrocytes. GJB6 gene (Cx 30) expression is under circadian control; it peaks during the dark period corresponding to the spontaneous waking period in mice (Maret et al. 2007) . Sleep deprived mice exhibited an increase in expression of GJB6 but not of GJA1 (Cx43) compared to control animals (Liu and Giaume unpublished data) . In addition to setting regulations directly related to sleep, glucose- and lactate-permeable astroglial gap junctions have been demonstrated to provide the basis for metabolic networks that regulates brain energy homeostasis and that sustain neuronal activity in hypoglycemic conditions (Rouach et al. 2008) . Moreover, Cx hemichannels in astrocytes have been shown to be permeable to glucose (Retamal et al. 2007) . Therefore, the reported alteration in the astrocyte-specific glycogen metabolism when wakefulness is prolonged instrumentally or pharmacologically (Petit et al. 2010) may be associated with changes in astroglial Cx-based channels since astroglial gap junctions provide the support for metabolic networking (Rouach et al. 2008) . Based on these observations, astrocytic Cx30 could be a candidate that exhibits changes in expression and function when the sleep-wake cycle is perturbed. As neuronal activity is altered through different phases of the sleep-wake cycle, this working hypothesis is strengthened by evidence demonstrating that Cx30 is regulated by neuronal activity. This is established at transcriptional level since GJB6 expression is increased in mice exposed to an enriched environment (Rampon et al. 2000) and at the functional level since gap junctional communication mediated by Cx30 channels is controlled by neuronal activity in the glomerular layer of the olfactory bulb (Roux et al. 2011) .
18.5.3 Drugs Affecting Sleep-Wake Status Have Differential Impacts on Cortical Astroglial Networks
One approach to investigate whether Cx gap junction channels are involved in sleep homeostasis is to determine whether Cx expression and function are affected by molecules that impact sleep and/or treat sleep disorders . One of the most prescribed molecules is modafinil, a eugeroic, which following administration in humans and mice suppresses both NREM and REM sleep . Compared to REM sleep and waking, a significant decline of energy metabolism is observed in cortical areas during NREM. This is shown using glucose uptake measurement in many animal species as well as in human (Ramm and Frost 1986; Vyazovskiy et al. 2008; Nofzinger et al. 2002) . Since NREM represents more than 85 % of the total sleep time in B6 mouse, it can be assumed that neurometabolic requirements are larger in modafinil-treated mice than in control mice (i.e. sleeping mice). Recently, this compound was tested for its effects on the expression of Cx43 and Cx30 at the mRNA and protein levels (Liu et al. 2013). This study performed in the cortex of adult mouse demonstrated that Cx30 levels increased, while those of Cx43 were not affected, after modafinil administration. In addition, in acute cortical slices, modafinil increases dye coupling in a dose-dependent manner and this effect is abolished following tetrodotoxin treatment in order to suppress neuronal activity (Liu et al. 2013). Interestingly, these observations are in line with a previous work carried out in thalamocortical slices demonstrating that gap junction-mediated electrotonic coupling is increased in neurons by similar concentration (150 μM) of and exposure time (30 min) to modafinil (Urbano et al. 2007) . As neurons express Cxs, Cx36 being a prevalent type (Söhl et al. 2005), these findings demonstrated that, in the somatosensory cortex, the general effect of modafinil on Cx-based intercellular communication is to enhance Cx-based networking in astrocytes as well as in neurons. Consequently, it was suggested that the wakefulness-promoting action of modafinil might be linked to an increase in neuroglial interactions. In addition, the effects of modafinil on gap junctional communication are prevented by carbenoxolone, an extensively used gap junction blocker (Beck et al. 2008) . In this study carried out in freely moving postnatal day 13 rats, the modafinil-induced increase of the sleep state-dependent, auditory-evoked potential amplitude was also blocked by carbenoxolone administration. In contrast, in acute cortical slices gamma-hydroxybutyric acid (GHB), a compound that has an effect on sleep regulation opposite to modafinil, has an inhibitory action on astroglial gap junctions, an effect which is not tetrodotoxin sensitive (Liu et al. 2013). This GHB inhibition observed with experiments carried out in acute cortical slices is in agreement with previous works performed in cultured astrocytes showing that sleep-inducing oleamide decreases gap junctional communication (Guan et al. 1997) .
However, it cannot be generalized that drugs maintaining wakefulness increase coupling while those inducing sleep decrease coupling. As illustrated in Fig. 18.3a, Ritalin® (methylphenidate), another molecule known to have a wakefulness-promoting action, inhibits gap junctional communication (Liu and Giaume unpublished data) . This discrepancy is likely due to different mechanisms of action. A similar remark is also true for the different sensitivity of the modafinil and GHB effects to tetrodotoxin.
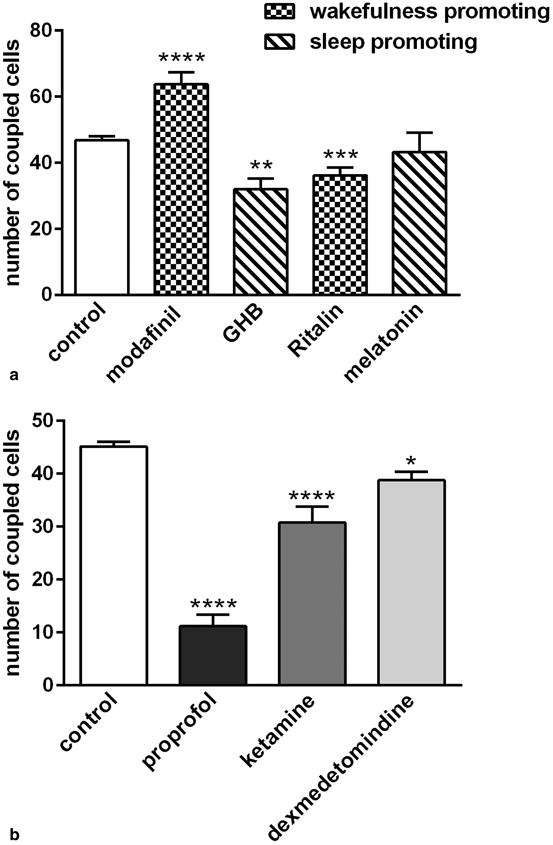
Fig. 18.3
Effects of drugs affecting the sleep-wake cycle and anesthetics on gap junctional communication between astrocytes in acute cortical slices. Gap junctional communication in astrocytes was assessed as previously described (Liu et al. 2013). a Effect of wakefulness-promoting drugs (200 μM modafil and 20 μM Ritalin®) and sleep inducing drugs (1 mM GHB and 4 μM melatonine) on the number of coupled cells after the whole-cell recording of a cortical astrocyte. b Differential inhibitory effect on astrocyte coupling exerted by three different classes of anesthetics used at clinical relevant concentration (150 μM for propofol, 300 μM for ketamine, 1 μM for dexmedetomidine)
18.5.4 General Anesthetics Inhibit Astrocytic Gap Junctional Communication and Hemichannel Activity in Acute Cortical Slices
Some general anesthetics, such as halothane and enflurane, have for a long time been considered as broad inhibitors of gap junctions (see Rozental et al. 2001) . Indeed, a screening of several classes of anesthetics has been performed using confluent primary cultures of mouse astrocytes (Mantz et al. 1993). This initial study revealed that the intravenous general anesthetics, propofol and etomidate, as well as halogenated anesthetics, halothane, enflurane and isoflurane, induced significant and dose-dependent inhibitions of gap junctional communication. In contrast, diazepam, morphine, ketamine, thiopental and clonidine were ineffective. We investigated whether their inhibitory action on gap junction channels could also be observed in acute cortical slices that are a more integrate model to study astrocyte properties. As illustrated in Fig. 18.3b using three different anesthetics, propofol, ketamine and the more recently developed dexmedetomindine, we also observed differences in the inhibitory potency when these drugs were used at clinically relevant concentrations (150, 300 and 1 µM, respectively). Indeed, as in cultured astrocytes propofol was found to be the most efficient, while ketamine had a significant effect in contrast to what was observed in culture, and dexmedetomindine had a weak but significant inhibitory effect. Again as stated previously for molecules affecting sleep status, there is not a general feature for the potency of effect of the tested anesthetics, although they all induce an inhibition of gap junctional communication in cortical astrocytes.
18.6 Concluding Remarks
An active and dynamic role is played by glial cells in many brain functions and pathologies (see Kettenmann and Ransom 2013) . Among glial cells, astrocytes are certainly one cell type that influences neuronal function and survival in both healthy and diseased conditions (see Giaume et al. 2007; Rossi and Volterra 2009; Verkhratsky et al. 2012; Parpura and Verkhratsky 2012) . We have largely focused this chapter on a single feature of astrocytes: the high expression level of Cxs. Astroglial Cxs are now associated with the modulation of synaptic activity and plasticity (Pannasch et al. 2011) , neuronal death (Froger et al. 2010; Orellana et al. 2011) as well as many other brain pathologies (see Giaume et al. 2010) . This is also the case for sleep-wake cycle regulation and its dysfunction, in which Cx channels could play a role. As neuronal behavior is characterized by synchronous network activities during sleep, it is expected that neuroglial interactions also involve interactions at an integrated level between neuronal circuits and astroglial networks. We have here discussed evidence indicating that several treatments known to affect sleep homeostasis have an impact on astroglial networking. In the near future we expect that evidence will emerge to demonstrate that sleep-wake cycle regulation is reciprocally affected by pharmacological or genetic modifications of Cx channel expression and/or functions in astrocytes. Hopefully, a definitive validation of the role of astroglial networks in sleep-wake cycle will contribute to the identification of valuable alternative therapeutic targets for the treatment of sleep disorders .
Acknowledgements
Works presented in this have been supported by the ANR grant AstroSleep NANR-12-BSV4-0013-01. The authors wish to thank Pr. P. Magistretti, Dr. J-M Petit, Pr. J. Mantz and Dr. S. Kandelam for helpful discussions related to Cx roles in the regulation of sleep-wake cycle .
References
Alanko L, Porkka-Heiskanen T, Soinila S (2006) Localization of equilibrative nucleoside transporters in the rat brain. J Chem Neuroanat 31:162–168PubMed
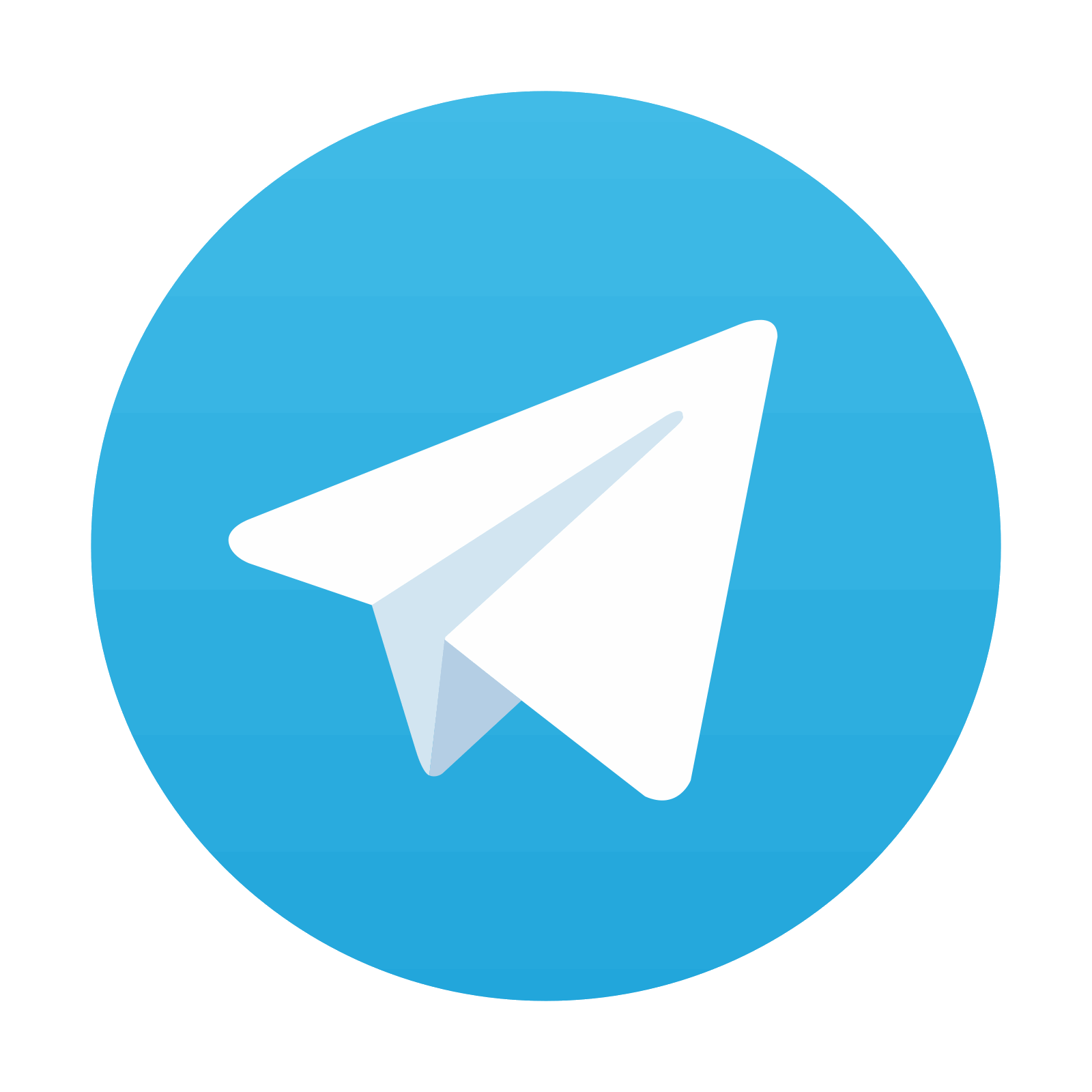
Stay updated, free articles. Join our Telegram channel
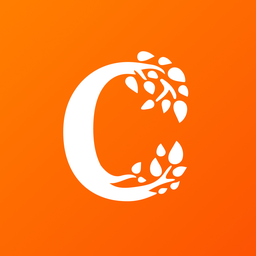
Full access? Get Clinical Tree
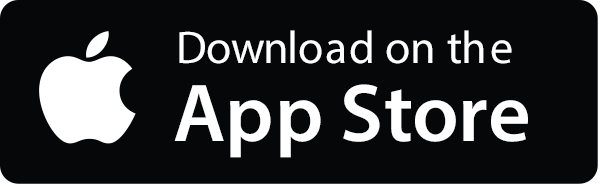
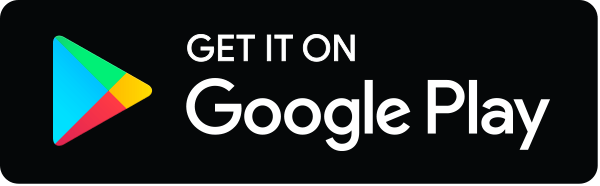