Introduction
Noninvasive monitoring of cerebral blood flow (CBF) among other aspects of neurologic function will add important tools to the armamentarium of the clinician. A noninvasive monitor could be applied to a much wider range of patients than existing intracranial pressure (ICP) monitors, which require that the patient be in an intensive care unit (ICU). It is now feasible to measure ICP with optic nerve ultrasound or with venous ophthalmodynamometry. Investigators have also combined ICP monitors with other noninvasive devices or used near-infrared spectroscopy to measure cerebrovascular pressure reactivity. The role of transcranial Doppler or color-coded duplex sonography that also can be used to noninvasively assess ICP and cerebral perfusion pressure is described in Chapter 30 . It also is possible that a noninvasive monitor could provide more physiologically relevant information about intracranial physiology (e.g., blood flow rather than blood pressure). This chapter describes one approach to this challenge, based on detection and processing of sound waves generated by the heart and transmitted through arterial vessels to the brain. Discussion of this brain acoustic monitor (BAM) begins with a description of the pathophysiology of brain injury and the physics of the cranium that make acoustic monitoring possible. Following is a discussion of how the BAM evolved to its present state, with evidence from a number of studies of its use in traumatic brain injury (TBI) and stroke. Illustrations of the output of the BAM as well as interpretation steps used to determine the existence of CBF anomalies are provided. A discussion of challenges and future directions of this technology concludes the chapter.
Pathophysiology of Detectable Brain Injury
The brain has the most highly regulated vasculature in the body. Neuronal function depends on aerobic metabolism, which in turn requires continuous delivery of oxygenated blood. Local autoregulatory processes act to maintain even blood flow and pressure within the brain across a wide range of systemic pressures. This autoregulation is exquisitely sensitive to disruption by TBI and other pathologies, but very difficult to study given the logistic constraints involved in use of the imaging tools, such as positron emission tomography (PET) scanning, in trauma or stroke patients in the emergency setting. Acoustics, however, offers an alternative approach to cerebrovascular monitoring that is less expensive and easier to apply early after injury.
Acoustics, the science of sound wave transmission, is commonly used in medicine to characterize the flow of blood and air through the body. The stethoscope, the very symbol of the physician, is one of the oldest and most useful of all medical devices. A stethoscope is a simple machine that amplifies naturally produced sound waves to an amplitude at which they can be detected and discriminated by the human ear. In this way physicians can examine blood flow through the heart and great vessels and airflow through the lungs. Although normal, laminar, flow is relatively inaudible, turbulence produced by obstructions or narrowing is clearly heard, and characterized variously as bruits, rales, wheezing, or the like. This is the principle that underlies the function of the BAM. Blood flow in the cranium is not audible to an external stethoscope, even with electronic amplification. The cranium does flex or vibrate in time to the arterial pulse, however, on a microscopic level. A highly sensitive contact sensor can be used to detect these vibrations and display them digitally. The BAM is thus an electronic stethoscope that “listens” to the blood moving through the brain and detects alterations in flow. Studies at the R Adams Cowley Shock Trauma Center of the University of Maryland have found that the brain acoustic signal the BAM measures is identical in morphology and component frequencies to the arterial pulse signal captured from any other artery in the body in normal patients, but substantially different following any trauma to the brain.
The observation of what the BAM measured provided the principal impetus for the study of this technology and evolved into assessing the signal itself and its characteristics as they differed in “normal” volunteers and patients with TBI. The process involved signal analysis in both time and frequency domains as is shown in the following examples, in which the brain-emitted signals are compared with those from a reference artery, such as the radial or digital artery. In the latest embodiment of the BAM system the metrics that indicate critical differences between reference and brain are coded into software that will identify anomalies in signals immediately. To broaden the basis of comparison, the BAM findings have been compared with computed tomography (CT) scan results, and with a group of indicators such as evidence of head insult, alteration of consciousness, Glasgow Coma Scale (GCS) score of less than 14, postconcussive symptoms, and general presentation. When these indicators are included, there is a high incidence of abnormal BAM scores in patients with evidence of TBI, compared with a very low incidence of abnormalities in normal volunteers.
Although in some ways this approach is novel, it is actually an extension of general auscultation with a visual display used instead of detection of audible sound differences. It is possible to amplify a BAM signal into the audible range, but the human ear is unable to detect the subtle differences in amplitude and frequency associated with TBI that become evident when a visual display is used to characterize the wave. Quantification of digitized data also makes it possible to track the progress of an individual patient over the course of treatment and recovery.
The diagnosis and subsequent clinical management of any patient with an acute brain injury depends on clinicians who integrate information from the patient’s history, from physical examination, from imaging studies, from laboratory tests, and from changes that occur over time. No one device can possibly provide an absolute black or white diagnosis in every patient. Instead the BAM is seen as an adjunctive technology that is simple, rapid, logistically compatible with the early care of trauma patients, and able to provide objective data on the quality of blood flow in the potentially injured brain.
Evolution of the Acoustic Monitoring Approach and Current Embodiment
Some notes on the somewhat serendipitous evolution of this approach will shed light on the mechanisms involved in brain acoustics. The study began with a visit from a group of U.S. Army Rangers to acoustic engineers now working at Active Signal Technologies. Their objectives were to determine if the science of exploring the ocean with sound could be used to explore the brain and to develop an ICP monitor the size of a cigarette pack—the space the rangers had for such equipment. The initial efforts following these discussions indicated that when small exciters at the temple interrogated the brain and the results were measured at the forehead (a system based on active sonar), variations in ICP would cause a difference in acoustic response. The approach encountered the challenge of calibrating this difference against absolute levels of ICP across different patients due to individual differences. During the course of this effort, a physician noted that the passive sensing itself generated waveforms on the data-gathering analyzer that resembled those of the arterial line displays on the patient monitors. Furthermore, there was a difference in the morphology of the signals between healthy patients (those admitted with decreased level of consciousness due to alcohol, but with no brain injury) and those with documented TBI and elevated ICP. Unlike the ICP values themselves, these differences appeared to be constant over a range of patients, at least at the basic level of those with and without TBI. Following this proof of principle study, further studies of in-hospital, prehospital and stroke applications of passive acoustic monitoring applied to cerebral and systemic blood flow have been conducted.
The Acoustic Science of Brain Monitoring
There is a limited literature about passive brain monitoring; the approach described here relies on the first principles of acoustics. Fundamentally, stethoscopes are air-coupled devices designed primarily to detect sounds generated in air-filled structures (the lungs). To better detect variations in brain sound emissions, sensors were built to “match” the brain, which is close in density to water. The sensor systems relied on “hydrophone” technology, instead of diaphragm-based air-coupled stethoscope technology. This approach was used because the cranial sensors were not immersed in the fluid of the brain, but rather “coupled” to the outside of the skull at a point anterior to the upper portions of the forehead above the sinus cavity. A similar approach was used to capture systemic arterial signals directly over the radial or digital artery.
In the application of these principles to monitoring, the first finding was that the resulting system did not detect audible signals from the brain, although in some cases the resolution of the system could be increased to detect anomalous responses (refer to Moretti and Pizzi’s discussion of the basic technology as applied to active and passive stethoscopy in high-noise environments ). Rather, the critical and subtle features of the signal that contained most of the information had to rely on a high-resolution digital display.
The second finding was that the brain signal was out of phase by nearly 180 degrees with signals that came from a location over the temporal artery, and from the reference sensor. This is a useful feature of this technology because it enables the monitoring staff to readily distinguish the brain signal from one that might come from the superficial vasculature over the forehead. This distinction is shown in Figure 47.1 . When there is uniform pressure from the arterial pulse wave entering the cranium, the side of the head follows the phase of the pulse, and the front of the head where the sensors are placed is receding. This is the general rule, although there are some exceptions based on anatomic variations among patients.


Initial Studies
The initial BAM evaluation took place in a study of 30 patients who were admitted to the ICU with severe TBI and invasive ICP monitors. The results of this study were based on the time-variant waveforms or “time-domain” signals collected. The original results proved promising and the analysis techniques moved to conventional time and frequency domain signal analysis. Further studies were performed in stroke patients and other environments including the admitting area of a trauma center to determine early response to brain insult and the prehospital environment. In addition studies have been performed to examine how BAM relates to conventional TBI metrics, and how the BAM tracked TBI effects over a period of time following the original incident.
Current Status
The BAM system continues to evolve (an example of a BAM used between 2005 and 2008 is shown in Fig. 47.2 ). The radial and finger sensor locations are shown, as well as the contact points of the head sensors. All sensors are held in place with bands except for the finger sensor, which is attached in much the same way as a pulse oximeter probe. To operate the system the researcher:
- 1.
Places the sensors on the patient
- 2.
Enters patient data in the acquiring laptop (or personal digital assistant [PDA])
- 3.
Activates the acquisition system, which records the sounds from all three locations, two head and one reference
- 4.
Checks the integrity of the signals
- 5.
Repeats if necessary until signals are representative
- 6.
Saves the data file and removes sensors and bands

This process normally takes approximately 5 minutes and can be performed without disrupting indicated clinical care (e.g., the study may be performed after initial physical assessment, while the patient is waiting for a CT scan). The BAM monitoring procedure also includes observing the autograding results of the system.
BAM Measurements in Normal Volunteers and Traumatic Brain Injury Patients
The BAM measures both the time variant amplitude of pulse waveforms and the frequency response of those waveforms. Examples are shown in the following text to illustrate the acoustic properties of normal subjects (controls) compared with those with TBI, beginning with data from a normal and a mild TBI patient shown in Figure 47.3 .



In Figure 47.3 A and D show the “time domain” signal in volts, representing the amplitude and direction of skull deflection in real time from two points and a reference. The green trace is from a sensor on the right forehead, the black trace is from a sensor on the left forehead, and the red trace is from the reference sensor over either the radial artery or the finger. In a non-TBI patient (see Fig. 47.3, A ), these signals are analogous, and morphologically similar, to the conventional trace produced by an arterial pressure catheter.
The system then performs a fast-Fourier transformation (FFT) on these signals that determines the component frequencies in the raw signal in a band from less than 1 Hz to 80 Hz—the frequency responses displayed in the middle of each panel (see Fig. 47.3, B and E ). In the absence of severe pathology, the highest peak in the frequency response should be the first one (the fundamental), which is determined by the arterial pulse rate. In a normal individual this should be around 72 beats per minute, or just greater than 1 Hz. The frequency responses of the 3 signals from the normal volunteer (see Fig. 47.3, B ) essentially overlap starting at the approximately 1 Hz fundamental and descending to the approximately 90 dB noise floor of the instrument, whereas the TBI patient’s brain responses (see Fig. 47.3, E ) diverge from the arterial reference greater than 20 Hz.
The current BAM system has a signal-processing algorithm that subtracts the frequency response of the artery from the brain after averaging, and highlights the divergence by calculating mean values of these relative frequency responses. The relative frequency responses of the left and right forehead signals are shown in panels of Figure 47.3 from 10 to 80 Hz—the band determined on the basis of correlation of critical frequencies within the arterial spectrum from both trauma and stroke patients. In normal individuals the morphology of the arterial pressure trace should be transmitted cleanly throughout the entire arterial tree, and so the relative frequency response of the brain (see Fig. 47.3, C ) should be a nearly flat line close to 0 dB. Indeed the normal relative frequency response curves in Figure 47.3, C are scattered around 0 dB and their mean values are close to 0 dB: left brain approximately minus 3 dB, right brain approximately minus 2 dB. Turbulence in the brain signal resulting from disrupted autoregulation will cause a discrepancy between the brain and systemic arterial frequency response, yielding a relative frequency response (see Fig. 47.3, F ) that rises to greater than 20 Hz.
Analysis Approach of Acoustic Response
Of the three principal metrics used for numerical assessment of the BAM signal, the first two (amplitude and ratio) are measured directly from the time domain signal (the raw data) and the third (divergence) is measured from the relative frequency response, which uses a systemic arterial control. These metrics are defined in the following text.
- 1.
Amplitude: An acceptable and representative amplitude is sometimes difficult to obtain for the brain because it is based on skull excursion for each arterial pulse and is only about 0.001 inch per heartbeat. The solid state sensors of the BAM can consistently measure a signal equal to about 0.1 V from positive to negative peak in a healthy normal subject and so amplitude less than 0.1 V is considered a positive indication for TBI. The systemic arterial reference signal is much stronger and consequently the system attenuates it in hardware or normalizes it in software so that it is consistently comparable to the brain signal.
- 2.
Ratio: The second metric is an initial crude assessment of signal morphology that calculates the peak excursion above the mean line divided by the peak excursion below the mean. Positive excursion should be at least twice as great as negative excursion and a ratio less than 2 is considered a positive indication for TBI. This is similar to the normal calculation of mean arterial pressure (MAP) in which the MAP is equal to (diastolic plus 1/3 [systolic minus diastolic]). Interestingly, this is a telling indicator. There can be some slight deviations, but the ratio cannot go below 2 positive to negative deflection to be safely considered normal.
- 3.
Divergence: The final and most important metric is derived from the frequency analysis. In a normal subject noise levels as indicated by the frequency-response slope should drop off rapidly at higher frequencies, whereas in the presence of brain trauma there will be higher amplitudes persisting into higher frequencies in the band. The divergence is defined as the maximum deviation of the brain signal’s relative frequency response above 0 dB. When the divergence is 10 dB or more, the patient is considered to have an anomalous brain noise compared to reference. In a mundane comparison, it is as if there is a quiet or laminar flow in the arteries when there is no disturbance, and an increase caused by some alteration of arterial tone causing disturbed flow (severely disturbed flow would be turbulent flow similar to that caused by a hose constriction).
All three metrics on both forehead sensors must register a “pass” to indicate a normal BAM; control and TBI patient BAM outputs are shown in Figure 47.3 . Here both the normal and mild TBI patient pass the amplitude criteria—both have amplitudes of at least 0.1 V in their brain signals bilaterally. However, the ratios of the normal are greater than 2 (see Fig. 47.3, A ) with both left and right brain signals exhibiting good pulsatile shape, whereas the brain-injured patient has ratios less than 2 and the brain signals are uneven—an unevenness not caused by artifact. The divergences of the normal brain signals in Figure 47.3, C are 7 and 8 dB for the right and left brain, respectively, indicating BAM negative for TBI, whereas the TBI signals have a maximum divergence of 20 dB (see Fig. 47.3, F , left brain), indicating BAM positive for TBI.
This analysis now has been automated, so that the results of the amplitude, ratio and divergence calculations appear on a pop-up panel with a color coding of yellow or green that indicate whether the person either is out of the bounds of the metrics established for controls or, in the case of green, that the person passes all criteria. In the event of a yellow, the screen shows the sensor(s) and metric(s) that are out of bounds and the procedure is to adjust the sensor position(s), band tension, and so on to accommodate for individual differences and retest. When the results appear to repeat (at least three times) they are considered representative, whether yellow or green, and the data are saved. In general, it is difficult to obtain a green reading when there is any anomaly in the patient’s blood flow, but yellow readings can often be corrected through adjustments to sensor placement. An example of these displays is shown in Figure 47.4 , in which the yellow reading is checked and the left brain sensor adjusted to yield a green reading with all metrics being within acceptable limits. The system now has evolved from initial time domain readings early in the study, to the later time and frequency domain data collection, comparison with a systemic arterial reference, and analysis with the autograding now in a clearly readable format.
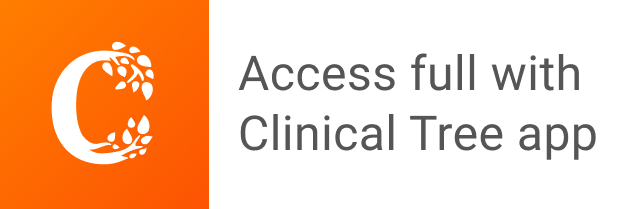