Introduction
Computed tomography (CT)–based imaging technology continues to be the principal imaging modality in patients who require neurocritical care. CT imaging can monitor intracranial events, CT angiography (CTA) can demonstrate intracranial and extracranial vasculature, and CT perfusion (CTP) can provide measures of intracranial blood flow. All of these studies are done on multislice CT scanners using commercially available software and can be performed on portable scanners housed in the neurocritical care unit (NCCU) (i.e., point-of-care assessment). Noncontrast head CT shows anatomic intracranial injury: hemorrhage, hydrocephalus, contusion, mass effect, shifts, ischemia, infarction, shear injury, and diffuse axonal injury (DAI). Contrast-enhanced head CT facilitates identification of medium to large vascular abnormalities (although typically not aneurysms), masses, and blood-brain barrier disruption. Contrast also is required for CTA and CTP. CTA may show vascular occlusion in a patient with an acute infarct, the source of an intraparenchymal hemorrhage, or vasospasm following subarachnoid hemorrhage (SAH). CTP is used to evaluate infarct core versus penumbra in a patient with an acute infarct, to show areas of reduced blood flow in a patient with vasospasm, and to demonstrate the presence or absence of autoregulation following traumatic brain injury (TBI).
A Brief Comparison with Other Techniques
Other imaging and monitoring techniques can provide similar information to that obtained by CT (See also Chapter 27 , Chapter 28 , Chapter 29 ). This information can complement or supplement the CT data. The presence of an ischemic infarct or the extent of injury with DAI is more accurately determined using magnetic resonance imaging (MRI). Disadvantages of MRI when compared with CT include the limitations in identification of acute intracranial hemorrhage, difficulty monitoring an unstable patient in the MRI suite because of requirements for nonferromagnetic life-support devices, longer imaging times with MRI, and the relatively greater expense of MRI equipment. The additional information from an MRI does not usually change acute management in patients with TBI. Magnetic resonance angiography (MRA) is a standard tool to image intracranial and extracranial vasculature in nontraumatized patients and is frequently used in patients with acute ischemic infarcts who also undergo MRI when being evaluated for urgent intra-arterial thrombolysis. In these patients magnetic resonance perfusion (MRP) is used to define areas of abnormal perfusion not yet infarcted (diffusion-perfusion mismatch). In addition, MRA can be useful to assess vascular dissections. However, MRA and MRP have the technical limitations of MRI in a patient on life support.
Cerebral perfusion also is measured with nonradioactive xenon-CT (Xe-CT). The advantage of stable Xe-CT is the possibility to measure regional cerebral blood flow in a quantitative manner. During a Xe-CT, xenon gas is inhaled in combination with oxygen over 5 minutes during CT scanning. Xenon is lipid-soluble and passes freely though the blood-brain barrier. Therefore brain, venous, and end-tidal xenon concentrations are assumed to be similar. Xenon is radiodense, and the density of xenon on a CT image has been shown to correlate with end-tidal xenon concentration. Calculation of cerebral blood flow (CBF) is based on use of the Fick equation. Accuracy of CBF measurement using stable xenon has been confirmed by comparison to radiolabeled microsphere studies. Xe-CT has some inherent limitations. Side effects of xenon inhalation include sedation, bronchospasm, and respiratory depression. Xenon blood flow measurements require specialized equipment to deliver the gas and to measure the end-tidal xenon concentration. Because the gas is sedating, some patients have difficulty lying still for the 5-minute scan acquisition. Availability of xenon gas is variable in the United States and at the time of publication is used under research protocols.
Transcranial Doppler (TCD) is used to evaluate cerebral perfusion by measurement of flow velocities in first- and second-order vessels. Access depends on adequate acoustic windows and accuracy may be operator dependent. An advantage to TCD is ease of use (typically bedside) and repeatability.
Single photon emission computed tomography (SPECT) using Tc-99m ethyl cysteinate dimer (ECD) or Tc-99m hexamethyl propyleneamine oxime (HMPAO) provides a qualitative evaluation of cerebral perfusion. The isotope is bound on the first pass through the brain. Evaluation of supratentorial flow is made in relation to cerebellar flow. Repeat evaluation cannot occur until after the technetium isotope has decayed.
Techniques
Computed Tomography (Nonenhanced CT)
Cross-sectional images of the head from a nonenhanced head CT scan show the major intracranial anatomy (ventricles, cisterns, sulci, gray matter vs. white matter borders) and the skull anatomy. A head CT demonstrates the presence of acute intracranial hemorrhage more reliably than any other imaging examination. Other pathologic processes that can be identified include midline shift, mass effect, hydrocephalus, intracranial edema, and encephalomalacia. Skull and facial fractures are shown from the same scan acquisition using windows and reconstruction algorithms maximized for bone. The only contraindication to a head CT is an inability of the patient to lie on the CT table. In the setting of acute intracranial decompensation, pregnancy or pediatric age become relative considerations or contraindications because CT requires the use of ionizing radiation. A head CT can be acquired in 1 to 2 minutes using current-generation scanners. Life support equipment, including intracranial monitoring systems, is easily accommodated. Scans are typically displayed in an axial projection but can be reconstructed into other planes, usually coronal or sagittal.
A head CT does not show vascular structures, may not show masses, may not easily aid identification of subacute-to-chronic hemorrhage, may not demonstrate demyelination, and may not identify intracranial infection. Small infarcts or new infarcts in patients with prior ischemic areas may be hard to visualize. Depending on the clinical question CT with iodinated contrast, MRI without or with gadolinium-based contrast, or vascular imaging using either CTA, MRA, or catheter angiography may be a more informative imaging procedure.
Computed Tomography Angiography
CTA scans follow the first pass of a contrast load as it moves through the vascular tree of the scanned anatomy. The scan acquisition is typically timed to be 1 to 2 seconds behind the arterial peak of the contrast bolus. The smallest available slice is used for the scan acquisition to improve detection of small vascular abnormalities and to improve the three-dimensional (3-D) reformations of the vascular volumes. Scans can be viewed as the initial acquisition (source images), as maximum intensity projections (MIPs) often thicker than the initial scan acquisition, or as true 3-D volumes. MIPs usually include the surrounding brain and bone. This is a disadvantage when evaluating the cavernous segments of the internal carotid arteries or the vertebral arteries within the foramen transversaria of the cervical vertebrae. MIPs facilitate visualization of longer segments of the vessel because the entire diameter of the vessel is included in the volume and MIPs can be obtained in any desired orientation. Intracranial vessels are well seen with a combination of coronal, axial, and sagittal projections. Extracranial carotid and vertebral arteries may be better evaluated using coronal, oblique, and sagittal reformations. Three-dimensional volume reformations eliminate the surrounding bone and brain and are rotated in space, either as single images or as a cine loop. Fuzzy connectedness also may be used to eliminate bony structures at the skull base. However, the diagnostic accuracy of bone-subtracted CTA often is no better than careful analysis of 3-D volume-rendered CTA when source data are assessed in multiplanar reformats.
Evaluation of CTA examinations should include the source images as well as the MIPs and the 3-D reformations. Source images show small vascular wall abnormalities. The reformations show the anatomic relationships of the vessels. Atherosclerotic narrowing of a vessel may be more easily appreciated on the source image and may be averaged out on an MIP or 3-D reformation. Conversely the caudal-pointing dome of an anterior communicating artery aneurysm or cranial-directed dome of a basilar tip aneurysm may be difficult to define on axial source images but will be clearly shown on the reformations. The common artifacts in a CTA examination arise from patient motion, which causes misregistration in the MIPs and in the 3-D reconstructions. An inadequate contrast bolus caused by a mistimed contrast injection or due to poor cardiac output can occur but is less common. Numerous studies have documented the accuracy of CTA for aneurysms greater than 4 to 5 mm in diameter. Aneurysms 3 mm or less are missed 20% of the time.
Computed Tomography Perfusion
Calculation of CTP parameters is based on the central volume principle, represented by the following equation:
CBF = CBV / MTT
where CBF equals cerebral blood flow (mL/100 g/min −1 ), CBV equals cerebral blood volume (mL/100 g), and MTT equals mean transit time (seconds, time of the bolus passing through a volume of tissue). One commonly used method uses deconvolution, a mathematical process, to derive these parameters from the time density curve generated by a first-pass measurement of contrast through the brain. MTT is calculated from the arterial input time-density curve, CBV is calculated as the area under time-density curve in the tissue of interest, and CBF is determined using the central volume principle. The density of the iodinated contrast is correlated to the volume of contrast in a region. Another CT-based technique uses a maximum slope model from this calculation. There is also variation between commercial vendors in the implementation of the deconvolution algorithm. This results in variability of the quantitative results from CT perfusion calculations. Older techniques to measure cerebral perfusion used tracers that were freely diffusible across the blood-brain barrier and not limited to the intravascular space (such as xenon in radioactive and nonradioactive forms). Iodinated contrast in contradistinction to xenon remains intravascular under normal circumstances. Actual perfusion of brain parenchyma is not shown. Although data obtained from Xe-CT cannot be directly applied to CTP due to technical differences, generally good correlation between CTP and Xe-CT is reported.
The advantage of CTP is widespread availability and relatively low cost. CTP can be obtained on any multislice CT scanner with appropriate software availability. A disadvantage of CTP is the limited anatomic coverage of the brain using 16- to 128-slice scanners. The length of the CT detector determines the volume of brain evaluated on a single CTP acquisition. Attempts to move the table during the CTP acquisition are difficult to implement and have not been generally adopted (toggle table). The posterior fossa is difficult to evaluate during a CTP study because of artifact from the bone in the skull base and because it is difficult to image the posterior fossa without scanning through the lens of the eye. Gantry angulation can eliminate the direct dose to the eyes while including the top of the cerebellum and the occipital lobes in the imaging plane. This angulation is usually not able to include the lower portions of the posterior fossa.
Although CTP is described as quantitative, postprocessing techniques can affect the measurements. Issues of input vessel selection, vessel opacification in the area being evaluated, and variation in acquisition software remain. Postprocessing can be done manually or automatically. Interobserver agreement in measurements of CBF, CBV, MTT and volume of infarct core and penumbra is better with automatically postprocessed data. CTP calculations also are based on the assumption of an intact blood-brain barrier. Many studies use internal comparisons to evaluate CBF, CBV, and MTT rather than the numerical values and reproducibility of quantitative assessment has been questioned, especially in processes such as acute ischemic infarct.
Blood flow in normal mixed gray and white matter is 50 to 80 mL/100 g brain tissue/min. CBF is higher in the gray matter compared with white matter. CBF is kept relatively constant by autoregulation mechanisms. In the absence of changes to the autoregulatory system CBF changes with alterations in PaCO 2 , functional requirements, and administration of vasoactive substances. Under normal conditions CBF is not changed by changes in systemic blood pressure. Low-flow states are of concern because of the risk of stroke. Nerve cell function deteriorates at CBF less than 20 mL/100 g/min. Cell death occurs at CBF less than 5-10 mL/100 g/min.
Patient Safety
Radiation dose and contrast load are significant patient safety issues with CT-based imaging. CTA scans are similar to other CT studies in that the scanner acquires single slices at multiple levels. The usual issue for these patients is the number of diagnostic cranial CT exams obtained per patient rather than the dose delivered per study.
CTP studies require acquisition of 40 to 50 scans over 1 minute at a single anatomic level. The scanning techniques used for a perfusion CT are lower than those for a diagnostic head study, typically 80 to 100 kVp/200 mA for CTP compared with 120 to 130 kVp/100 to 250 mA for a head CT. The lower kVp of CTP results in a lower radiation dose and improved visualization of the iodinated contrast. Radiation dose measures ionizing energy absorbed per unit of mass and is measured in gray (Gy) or milligray (mGy); 1 Gy = 1 joule per kilogram. Alternative units are sieverts (Sv) or millisieverts (mSv). For x-ray radiation in the ranges produced by CT scanners, 1 mSv = 1 mGy. When CTP is done at the basal ganglia level, avoiding the orbits, intracranial radiation dose is 180 to 363 mGy, surface dose is 327 to 713 mGy, and lens dose (from scatter) is 11 m to 22 mGy. This is higher than the average surface dose of 66 to 83 mGy at the tuber frontale by conventional head CT scan using 130 to 135 kVp and 105 to 270 mA. If CTP is performed at multiple levels, the dose is increased in proportion to number of acquisitions. The threshold dose for cataract formation is 2 to 10 Gy. The overall effective dose equivalent for CTP is 2 plus or minus 2.5 mSv. The dose from a CTA of the brain is 2 to 4 mSv. The dose from other blood flow measurement techniques, such as positron emission tomography (PET) and single photon emission computed tomography (SPECT), is higher. For comparison, background radiation dose over a year in approximately 3 mSv, the dose from a chest x-ray is 0.1 mSv, and the dose during a roundtrip flight from Los Angeles to New York is 0.03 mSv.
Iodinated contrast intravenously administered increases serum creatinine. Patients with compromised renal function may be at greater risk. CTA usually requires 60 to 100 mL of contrast, CTP 40 to 50 mL per anatomic level evaluated. The significance of these contrast doses depends on the patient’s underlying renal function, total amount of contrast given, and the timing of those doses. For example, if a patient has an acute SAH, the following studies may happen in rapid succession: CTA and diagnostic catheter angiography without or with aneurysm coiling, each with a significant dye load. In a patient admitted for polytrauma, admission studies may include CTA of the cranial and extracranial cervical vessels, CTA of aorta and peripheral vessels, abdominal CT with contrast to evaluate for abdominal injury, and possibly cranial CTP to evaluate the presence of absence of cerebral autoregulation. A patient with an acute infarct is likely to have a more limited set of studies: CTA and CTP. Only the minority who might benefit from intra-arterial treatment are likely to have angiography. Additionally, in a stroke patient, MRI, MRA, and MRP may be used acutely, possibly diminishing the volume of iodinated contrast. This is, however, the patient population most likely to have compromised renal function secondary to systemic processes such as diabetes and hypertension.
Indications
Routine Head Computed Tomography Scan
The indications for noncontrast head CT are well established and described, and the reader is referred to recent reviews or textbooks that describe this it in detail. Here is provided a brief synopsis of how head CT can aid the neurointensivist.
A head CT is the initial emergent study for patients with acute neurologic injury, whether stroke, trauma, confusion, or unknown neurologic event. It also is the primary study to follow the neurologically compromised patient in an intensive care unit (ICU) setting. A typical example is the patient with an acute intracranial hemorrhage. The acute and subacute management questions can involve resolution of the hemorrhage with diminishing mass effect, increasing parenchymal vasogenic edema in response to intraparenchymal blood products, increased volume of blood if delayed hemorrhage occurs, or hydrocephalus if blood within the arachnoid granulations causes diminished cerebrospinal fluid (CSF) resorption. Other issues addressed by head CT include identification of generalized brain swelling such as might occur in a patient with DAI, midbrain compression (unilateral from uncal herniation or bilateral from diffuse swelling), midline shift, new or evolving brain infarction, postoperative issues, or new fluid collections. Identification of possible infection or vascular injury requires a contrast study.
Imaging Appearence
Intracranial Hemorrhage
Acute intraparenchymal hemorrhage is denser than brain with CT. The density reflects the concentration of the protein component of the hemoglobin molecule and diminishes with breakdown of the hemoglobin molecule. An uncomplicated clot within the brain becomes more lucent on its margins, continues to decrease in density over time (2-3 weeks depending on the initial size of the clot), and ultimately resorbs, leaving an area of encephalomalacia (low density with focal volume loss). When contrast is given, contrast extravasation is associated with subsequent intracranial hemorrhage growth. This is similar to the “spot sign,” a bright spot on CTA source images that predicts hematoma growth ( Fig. 26.1 ).

SAH, unlike intraparenchymal hemorrhage, remains within the sulci and cisterns. It does not undergo the breakdown process seen with intraparenchymal clots. Density is dependent on the concentration of blood in the subarachnoid space (i.e., the volume of the hemorrhage within the volume of CSF). Small hemorrhages may be poorly seen because of mixing of the blood with CSF. Resolution of subarachnoid blood is dependent on clearing by CSF circulatory processes. Attempts have been made to quantitate the volume of SAH in relation to the likelihood of developing vasospasm. The Fisher scale was an early method to correlate the volume and distribution of subarachnoid blood to the development of vasospasm. Subsequent modifications or the development of newer grading scales have improved the predictive reliability. In the Hijdra scale the volume of blood in each of 10 basal cisterns and four ventricles is separately evaluated and summed for a total score.
Acute blood within a more chronic fluid collection may be seen as a blood-fluid level with the denser blood (both in terms of visual appearance and true fluid density) in a dependent part of the collection. This often is seen as blood in the occipital horns (blood layering in the dependent part of the ventricular system in a supine patient), but also occurs in chronic subdural hematoma (SDH) and in other cystic cavities.
Acute Subdural Hematoma
Acute SDH is found within the subdural space, superficial to the arachnoid. On imaging, acute SDH conforms to the curvature of the skull, is confined by dural boundaries (does not cross the midline), may extend between the leaves of the dura in the falx and tentorium, and may extend across suture lines. An acute SDH tends to be higher density than brain. Chronic SDH is of lower density than brain because of hemoglobin breakdown similar to the process in intraparenchymal hemorrhage. Acute bleeding into a chronic SDH results in mixed density and blood-fluid levels. More chronic SDH may not conform as clearly to the curve of the skull.
Acute Epidural Hematoma
An acute epidural hematoma (EDH) is hyperdense to brain, bows away from the curve of the skull (sometimes described as lens shaped), and does not cross sutures but can cross the midline (crosses dural sinuses). An EDH often is associated with skull fractures, classically in the squamous temporal bone across the path of the middle meningeal artery. EDHs, which lie superficial to dura, are less likely than SDH to be associated with an adjacent brain contusion.
Increased Intracranial Pressure
Increased intracranial pressure (ICP) causes a decrease in the size of CSF spaces in the brain followed by increasing severity of herniation. Diagnostic clues include a generalized decrease in the size of sulci and cisterns. In particular, compression or absence of the perimesencephalic cisterns is an indication that ICP may be elevated. However, head CT findings do not always reliably predict intracranial physiology (e.g., ICP and brain oxygen). If the increase in ICP is due to diffuse swelling, the ventricles also may decrease in size. If it is due to hydrocephalus, the ventricles may increase in size. When it is associated with local mass effect and herniation, there may be compression of the ventricles on the side of the lesion, displacement of midline away from the lesion, and effacement of cisterns. An example is subfalcine and uncal herniation in a patient with a subacute, large, middle cerebral artery infarct with marked cytotoxic edema.
Herniation
The appearance of herniation depends on the location of the mass lesion or mass effect within the brain. Uncal herniation and subfalcine herniation occur with mass lesions on one side of the brain ( Fig. 26.2 ). The uncus is displaced toward the opposite side of the brain from the intracranial mass, the suprasellar cistern is distorted, and the midbrain is displaced away from the mass and compressed. Subfalcine herniation is usually part of this constellation with compression of the ipsilateral ventricle, compression of the third ventricle, and displacement of the third ventricle and septum pellucidum across midline away from the mass lesion. In general, when there is more than 5 mm of midline shift, a surgical solution for the increase in ICP often is necessary. Uncal herniation can be bilateral. This might occur if the patient had diffuse swelling. In this case all supratentorial ventricles are compressed, the suprasellar cistern and perimesencephalic cisterns are effaced, and the midbrain is flattened.

Posterior fossa mass effect can be seen as compression and displacement of the fourth ventricle, compression of the cerebellopontine-angle cisterns and cisterns anterior to the brainstem, and displacement of the vermis upward with flattening and distortion of the quadrigeminal plate cistern (tectum) (i.e., “upward herniation”; Fig. 26.3 ). Also possible, but more difficult to appreciate using CT, is downward displacement of the cerebellar tonsils into the foramen magnum.

Infarct
Ischemic injury to the brain on CT ( Fig. 26.4 ) is seen as areas of low density with poor to absent identification of the difference between gray matter and white matter (loss of gray-white differentiation). Examples are the insular ribbon sign with absence of a visible insula on the CT scan, generalized loss of gray-white differentiation in an arterial vascular territory, or loss of gray-white differentiation in the hemispheres in a patient with diffuse anoxic injury. Acutely there may be minimal mass effect. With time (12-48 hours) there can be marked swelling of the areas of cytotoxic edema with possible herniation. Occasionally hemorrhagic transformation (i.e., the development of hemorrhage within an acute or subacute infarct) may occur.

The appearance of a venous infarct differs from that of an arterial infarct in the distribution of the low-density region and the propensity toward hemorrhage. The areas of low density do not correspond to conventional arterial distributions but may involve deep structures, the temporal lobe, and paramidline structures. The diagnosis depends on appreciating high density in a venous sinus, absence of contrast within a venous sinus on a contrast-enhanced CT (delta sign), or absence of a sinus on a magnetic resonance venogram ( Fig. 26.5 ). Venous sinus thrombosis is more reliably diagnosed than is the thrombosis of a superficial vein because of the variation in the distribution of superficial cerebral veins.

Computed Tomography Angiography
Indications for CTA include diagnosis and evaluation of intracranial aneurysm, either ruptured or unruptured, diagnosis of cerebral vasospasm, diagnosis of intracranial vascular malformations, evaluation of extracranial and intracranial vascular structures in a patient with ischemic infarct or chronic cerebrovascular disease, evaluation of underlying pathology in a patient with acute intracranial hemorrhage, diagnosis of vascular injury following blunt or penetrating trauma, and diagnosis of cerebral venous thrombosis (CVT).
Computed Tomography Perfusion
CTP is a developing technology, and its use is still to be fully elucidated. Indications include diagnosis of acute infarction, evaluation of patients with acute infarction for possible intervention, diagnosis of vasospasm, assessment of autoregulation in TBI patients, evaluation of blood-brain barrier permeability in acute infarction to assess for the risk of hemorrhagic transformation, measurement of cerebral vascular reserve in patients with chronic vascular occlusions, and evaluation of tumor vascularity.
Subarachnoid Hemorrhage
The sensitivity of noncontrast-enhanced CT for SAH within 12 hours of ictus is 98% to 100%. If there is a high suspicion for SAH or if the patient has a subacute hemorrhage, lumbar puncture may still be required to exclude the uncommon but possible false-negative CT. Some suggest when there is strong clinical suspicion of SAH that the combination of CT and CTA may offer a less invasive diagnostic paradigm than lumbar puncture. The quality of CTA to detect aneurysms is almost comparable to digital subtraction angiography (DSA). However, DSA remains superior to CTA to detect small aneurysms (<5 mm), especially with 3-D rotational angiography. Three-dimensional rotational angiography also allows for more precise evaluation and measurement of aneurysms. CTA can assist in the rapid diagnosis or exclusion of aneurysms and arteriovenous malformations in the acutely ill patient, such as the patient with a large intracerebral hematoma who is rapidly deteriorating. When used as a first screening test for intracranial hemorrhage, CTA is often as accurate as DSA for more proximal lesions but has a lower sensitivity for smaller more distal lesions and dural arterio-venous fistulas.
CT angiography can be used in the initial work-up of patients with a suspicion of an intracranial aneurysm ( Fig. 26.6 ). Romijn et al. compared CTA with bone elimination to DSA and 3-D rotational angiography in 108 patients with clinically suspected SAH. Specificity, sensitivity, positive predictive value, and negative predictive value of CTA with bone removal was 0.99, 0.90, 0.98, and 0.95, respectively. Sensitivity was 0.99 for aneurysms greater than or equal to 3 mm and 0.38 for aneurysms less than 3 mm. Other authors have found similar results without bone removal. When CTA is negative the false-negative rate is reported by some to be zero after DSA. In particular, negative CTA findings are reliable to exclude an aneurysm with a perimesencephalic distribution of blood. When the hemorrhage is diffuse or more peripheral, DSA should be considered when CTA is negative. However, the sensitivity of on-call resident interpretation of a CTA after SAH is lower than an attending, and among patients who undergo subsequent digital subtraction angiography there is a treatment plan change in about 20%. CTA may be used to decide whether to occlude the aneurysm using endovascular or surgical techniques, and in some cases, guide operative management. The need for catheter angiography before decisions of management (coil vs. clip) and to determine surgical approach are a balance between the immediate surgical concerns, the requirement for rapid intervention, and the experience of the involved physicians. Discrepancies between CTA and DSA frequently are associated with location, for example, cavernous vs. supraclinoid, or differentiation of a vascular loop from a small aneurysm. Use of CTA to evaluate aneurysms after occlusion (clip or coil) is less clear. The artifact from the metal coil or clip can prevent accurate visualization of possible residual aneurysm. Despite this, there are reports of CTA use to evaluate treated aneurysms with 87% to 97% sensitivity and specificity, although intraobserver concordance was less robust.
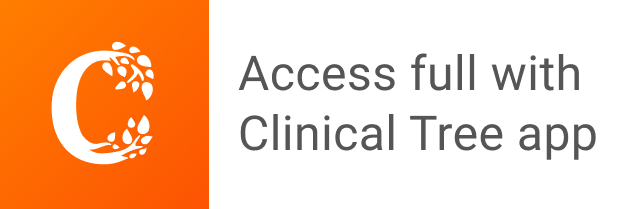