Subarachnoid hemorrhage from the rupture of a saccular aneurysm is a devastating neurological disease that has a high morbidity and mortality not only from the initial hemorrhage, but also from the delayed complications, such as cerebral vasospasm. Cerebral vasospasm can lead to delayed ischemic injury 1 to 2 weeks after the initial hemorrhage. Although the pathophysiology of vasospasm has been described for decades, the molecular basis remains poorly understood. With the many advances in the past decade in the development of sensitive molecular biological techniques, imaging, biochemical purification, and protein identification, new insights are beginning to reveal the etiology of vasospasm. These findings will not only help to identify markers of vasospasm and prognostic outcome, but will also yield potential therapeutic targets for the treatment of this disease. This review focuses on the methods available for the identification of biological markers of vasospasm and their limitations, the current understanding as to the utility and prognostic significance of identified biomarkers, the utility of these biomarkers in predicting vasospasm and outcome, and future directions of research in this field.
Subarachnoid hemorrhage (SAH) from the rupture of saccular aneurysms (nontraumatic SAH) is a devastating and often fatal disease. Its incidence is 6 to 8 per 100,000 people years. Even with the advancement and increased availability of neurosurgery and intensive care, the morbidity and mortality from aneurysmal SAH remains high. Prior to hospitalization, 12% of those with SAH die while approximately 40% of those surviving to hospitalization do not survive the first 30 days. These figures contribute to an estimated case-fatality rate of 25% to 50%. The most common cause of death after survival of the initial hemorrhage is vasospasm. SAH is most frequently related to trauma. However, it is rarely a singular cause of fatal outcomes in traumatic brain injury and is not commonly associated with the medical complications of SAH, although this has been debated recently. SAH from intracranial vascular rupture can be classified into two groups: those resulting from the rupture of a berry or saccular aneurysm, which represent 80% of nontraumatic SAH, and those associated with vascular malformations, such as arterial venous malformations, which represent 20% of all nontraumatic SAH. The classical medical complications associated with SAH, such as vasospasm and cerebral salt wasting, are usually associated with the rupture of saccular aneurysms and are further discussed in this article.
Vasospasm is the most common complication of SAH that leads to clinical deterioration. However, the molecular basis and pathophysiology are still poorly understood. Up to 70% of patients who survive SAH develop signs of vessel narrowing on transcranial Doppler ultrasound or angiography while clinically symptomatic vasospasm occurs in only 46% of those patients. Rates of infarction after SAH are estimated to be approximately 25%. Vasospasm can be defined both angiographically and clinically. Angiographic definition requires visualization of vessel narrowing in the affected vessel by angiography, while clinical vasospasm requires observation of the physical signs and symptoms of vasospasm. Classic symptoms of clinical vasospasm include altered levels of consciousness, confusion, focal motor symptoms, and new-onset aphasia. These symptoms typically develop over a period of hours to days and are referred to as delayed ischemic neurological deficits (DINDs). In the early days of subarachnoid surgery, most of the delayed neurological deficits were attributed to progressive cerebral edema from the initial damage of the hemorrhage or from the surgery itself. However, as time passed, it has become clear that DINDs are occurring independently of the initial hemorrhage or surgery.
The proximal event leading to DIND still remains unclear. However, two facts are well established: (1) DIND is the result of tissue ischemia and represents a form of ischemic stroke and (2) DIND occurs in vascular territories where vasoconstriction has been documented angiographically. These observations strongly suggest a causal relationship between vascular constriction and ischemia, although in recent studies it has become less clear that the primary event causing ischemia and cell death is the constriction of the associated blood vessel. It is possible that vascular constriction may be an epiphenomena or a contributing factor to parenchymal destruction and that the final ischemic event may be caused by other factors occurring directly within the parenchyma at the level of the neurovascular unit. This can be loosely supported by the results of trials, such as the Neurosurgery Cooperative Trial, which used the calcium channel blocker nicardipine, and the CONSCIOUS-1 Trial (Clazosentan to Overcome Neurological Ischemia and Infarction Occurring after Subarachnoid Hemorrhage–1 Trial), which tested the endothelin antagonist clazosentan. While both nicardipine and clazosentan prevented vasospasm, neither was shown to prevent ischemia or DIND. Mechanical intervention with angioplasty, though successful in reversing the narrowing of the vessel, also failed to prevent DIND when performed at the onset of symptoms or prophylactically before the symptoms develop. These interventions demonstrated efficacy in reducing the occurrence of angiographic vasospasm. However, they did not reduce the incidence of DINDs.
The failure of new drugs and techniques to protect against DINDs has spurred research to isolate proteins that may affect the development of vasospasm. These proteins represent potential therapeutic targets as well as markers of vasospasm onset. The use of proteomics and translational research to understand vasospasm has a long history and understanding the current techniques can aid clinicians interested in furthering vasospasm research or in applying the newest clinical techniques to aid in the care of patients with vasospasm from aneurysmal SAH.
Basics of the human proteome
Human proteomics is the study of human proteins in the context of three realms: drug discovery, proteome mapping, and biologic understanding. A number of new scientific techniques have been developed that allow for the identification of proteins from a number of human biologic tissues. This material, usually collected in a specific clinical context, gives researchers a glimpse of the activity occurring as the direct result of disease. The study of proteomics in vasospasm and DIND could potentially help identify a variety of biomarkers from diverse tissue sources, including serum, cerebrospinal fluid (CSF), and extracellular parenchymal fluid. Vasospasm is a molecularly based phenomenon that involves complex cellular signaling. The identification of proteins associated with different stages of disease progression may enable the isolation of specific protein signals. The understanding of vasospasm through proteome analysis requires data collected in the setting of well-described clinical phenotypes.
The characterization of the human genome has produced a wave of information that has made it possible to estimate the number of genes contributing to the human proteome. Thus far, 19,438 genes have been identified with an additional 2188 predicted genes based on data from the completed human genome study. Thus far, 34,124 transcripts have been isolated, contributing to more than 100,000 proteins. Each gene can code for an estimated 10 to 20 different protein species. Thus the human proteome is vast with many potential targets.
Tissue sampling
During the treatment of aneurysmal SAH, various parenchymal sites can be harvested for proteomic analysis. These include solid tissue, such as brain parenchyma; tissue approximating the vascular substructure, such as adventitia; and sections of vessel affected by aneurysmal rupture. Each of these tissue types requires sacrifice in the setting of surgical intervention and is rarely obtained in normal practice. More commonly acquired and easily accessible tissue types include blood, CSF, and extracellular fluid from cerebral microdialysis. Blood is a tissue with some characteristics of fluid. However it is actually a complex conglomerate more similar to that of a colloidal suspension. It potentially contains protein or genetic material from any compartment of the human body. Its proteome has not yet been completely characterized and is probably the largest of any tissue compartment in the body. The most commonly analyzed portion of human blood is serum and within it are tens of thousands of analytic targets. Injury or changes in any compartment of the human body are usually reflected in the proteome of blood. Thus blood is the most commonly accessed source for protein analysis in any clinical scenario.
CSF and extracellular fluid are the next two most commonly tested sources of body fluid for protein analysis in the setting of SAH. CSF studies of proteins in the context of vasospasm are relatively common. The protein concentration of CSF is 400 times less than blood. About 2600 proteins have been identified in CSF at this time and a proteome map of CSF is available from several sources ( http://www.expasy.ch/ ). Extracellular fluid is often difficult to obtain as it requires the placement of a cerebral microdialysis catheter and yields only small amounts of fluid. However, its use is more frequent now as microdialysis catheters are increasingly placed for monitoring ischemic stress in the setting of brain injury. Studies looking at extracellular fluid in the setting of SAH and vasospasm are still limited while study of the extracellular fluid proteome is incomplete. A number of peptides have been identified by mass spectroscopy and these peptides represent 27 individual proteins. All of these proteins appear to represent proteins derived from CSF.
Proteins isolated from blood exist in a background of great protein diversity represented by vast numbers of different protein types. It is estimated that 22 proteins represent 99% of the protein content of the blood. Thus, protein concentration in blood varies extensively, spanning 10 orders of magnitude. This dynamic range makes detection of a low concentration of small proteins specifically related to a single injury quite difficult. The dynamic range of mass spectroscopy, the most common means of identifying proteins in blood, is 2 orders of magnitude, not the 10 required to encompass the proteome. The situation is further complicated in that the proteins of highest interest tend to be those occurring at lower concentrations and are not the common constituents of blood. The solution to this problem requires both protein isolation and concentration with the goal of the purification and selection of specific components of blood.
The problem of dynamic range exists with CSF and extracellular fluid to a much lesser degree. The decreased number of proteins and the relative lower total concentration of protein lead to a decrease in the dynamic range and makes for more efficient protein isolation and concentration. However, there is still a wide dynamic range and the challenge of detecting proteins of interest in small samples with very low concentrations is still a major roadblock, particularly with the present technology.
Protein isolation
The first stage of proteome analysis requires the isolation of individual proteins. The most commonly applied technique for protein isolation is two-dimensional gel electrophoresis. This technique uses a two-stage process, including pH immobilization with isoelectric focusing, followed by standard polyacrylamide gel electrophoresis. The proteins can then be visualized using special stains or immunoblotting techniques. Other important protein isolation technologies include affinity chromatography, ion-exchange chromatography, reversed-phase liquid chromatography, and capillary electrophoresis. Chromatography using all mediums is a popular method to isolate proteins. Such techniques as high-performance liquid chromatography are common and effective. Isotope-coded affinity tagging is another technique that modifies liquid or gas chromatography to isolate proteins. Isotope-coded affinity tagging incorporates a thiol-specific reactive group, with a radioactive marker containing an affinity tag, such as biotin. These markers bind to predetermined protein moieties in specific proteins, enabling isolation with traditional methods, such as high-performance liquid chromatography. Other gel-free proteomic technologies, such as capillary liquid chromatography and capillary electrophoresis, enable the isolation and identification of extremely small protein samples in a liquid or gas state.
Tissue sampling
During the treatment of aneurysmal SAH, various parenchymal sites can be harvested for proteomic analysis. These include solid tissue, such as brain parenchyma; tissue approximating the vascular substructure, such as adventitia; and sections of vessel affected by aneurysmal rupture. Each of these tissue types requires sacrifice in the setting of surgical intervention and is rarely obtained in normal practice. More commonly acquired and easily accessible tissue types include blood, CSF, and extracellular fluid from cerebral microdialysis. Blood is a tissue with some characteristics of fluid. However it is actually a complex conglomerate more similar to that of a colloidal suspension. It potentially contains protein or genetic material from any compartment of the human body. Its proteome has not yet been completely characterized and is probably the largest of any tissue compartment in the body. The most commonly analyzed portion of human blood is serum and within it are tens of thousands of analytic targets. Injury or changes in any compartment of the human body are usually reflected in the proteome of blood. Thus blood is the most commonly accessed source for protein analysis in any clinical scenario.
CSF and extracellular fluid are the next two most commonly tested sources of body fluid for protein analysis in the setting of SAH. CSF studies of proteins in the context of vasospasm are relatively common. The protein concentration of CSF is 400 times less than blood. About 2600 proteins have been identified in CSF at this time and a proteome map of CSF is available from several sources ( http://www.expasy.ch/ ). Extracellular fluid is often difficult to obtain as it requires the placement of a cerebral microdialysis catheter and yields only small amounts of fluid. However, its use is more frequent now as microdialysis catheters are increasingly placed for monitoring ischemic stress in the setting of brain injury. Studies looking at extracellular fluid in the setting of SAH and vasospasm are still limited while study of the extracellular fluid proteome is incomplete. A number of peptides have been identified by mass spectroscopy and these peptides represent 27 individual proteins. All of these proteins appear to represent proteins derived from CSF.
Proteins isolated from blood exist in a background of great protein diversity represented by vast numbers of different protein types. It is estimated that 22 proteins represent 99% of the protein content of the blood. Thus, protein concentration in blood varies extensively, spanning 10 orders of magnitude. This dynamic range makes detection of a low concentration of small proteins specifically related to a single injury quite difficult. The dynamic range of mass spectroscopy, the most common means of identifying proteins in blood, is 2 orders of magnitude, not the 10 required to encompass the proteome. The situation is further complicated in that the proteins of highest interest tend to be those occurring at lower concentrations and are not the common constituents of blood. The solution to this problem requires both protein isolation and concentration with the goal of the purification and selection of specific components of blood.
The problem of dynamic range exists with CSF and extracellular fluid to a much lesser degree. The decreased number of proteins and the relative lower total concentration of protein lead to a decrease in the dynamic range and makes for more efficient protein isolation and concentration. However, there is still a wide dynamic range and the challenge of detecting proteins of interest in small samples with very low concentrations is still a major roadblock, particularly with the present technology.
Protein isolation
The first stage of proteome analysis requires the isolation of individual proteins. The most commonly applied technique for protein isolation is two-dimensional gel electrophoresis. This technique uses a two-stage process, including pH immobilization with isoelectric focusing, followed by standard polyacrylamide gel electrophoresis. The proteins can then be visualized using special stains or immunoblotting techniques. Other important protein isolation technologies include affinity chromatography, ion-exchange chromatography, reversed-phase liquid chromatography, and capillary electrophoresis. Chromatography using all mediums is a popular method to isolate proteins. Such techniques as high-performance liquid chromatography are common and effective. Isotope-coded affinity tagging is another technique that modifies liquid or gas chromatography to isolate proteins. Isotope-coded affinity tagging incorporates a thiol-specific reactive group, with a radioactive marker containing an affinity tag, such as biotin. These markers bind to predetermined protein moieties in specific proteins, enabling isolation with traditional methods, such as high-performance liquid chromatography. Other gel-free proteomic technologies, such as capillary liquid chromatography and capillary electrophoresis, enable the isolation and identification of extremely small protein samples in a liquid or gas state.
Protein identification
The time and effort required to identify proteins are determined in part by the abundance of the target of interest, as well as its purity, size, and stability during the purification process. The most commonly employed technique for definitive protein identification is mass spectrometry. Mass spectrometry employs protein ionization technology to produce ionized peptide fragments, from which mass-to-charge ratios are determined. Proteins are first eluted from a reversed phase column and then placed into the mass spectrometer. This is followed by chemical ionization and a mass-to-charge ratio determination for all of the individual peptides in the mixture of proteins. The tandem mass spectrometry technique is also frequently employed in protein identification. Individual ionized particles are isolated in time or space and then further fragmented by ionization. The resulting ionized products are further analyzed for their mass-to-charge ratio, resulting in a spectra, or collection, of masses for a given sample. The resulting spectra can then be compared to data from a large library of previously identified proteins. Most mass spectrometers can process approximately 7000 spectra per hour yielding identifiable proteins 10% of the time, enabling the identification of up to 700 to 1400 proteins an hour.
Variations of quantitative mass spectroscopy methods have been developed, each with its own advantage and disadvantage. The most common types are single or double mass spectroscopy, as previously discussed. However, this technique is limited in that it can isolate and identify only a few proteins from a given sample. Other mass spectroscopy techniques include electrospray ionization, which incorporates a coulombic pressure methodology to isolate single ionized columns of macromolecules in the setting of an aerosolized mixture. This enables greater protein isolation. Fourier transform ion cyclotron resonance mass spectrometry is another highly accurate mass spectroscopy technique. Especially suitable for samples with extremely low concentrations, this technique examines the cyclotron frequency of the charged macromolecule in a fixed magnetic field to determine mass-to-charge ratios.
For high-throughput protein analysis, approaches to proteome analysis now combine protein isolation with protein identification via mass spectroscopy. These methods can be used in situations in which the tissue examined has a large dynamic range. Surface-enhanced laser desorption/ionization–time-of-flight (SELDI-TOF) mass spectrometry isolates proteins by using a stage that incorporates protein binding to a surface plate. To selectively immobilize the target to the surface plate, this plate can be coated with antibodies or other substrates that interact with the sample. The surface is then energized with a laser and the ionized proteins are passed through an electric field and the time of flight is measured, which can be used to determine the mass-to-charge ratio. This method enables mass spectroscopy signaling and often direct protein identification. This technology can be used to produce signatures that incorporate mass spectroscopy and time-of-flight ion analysis, which enables the identification of unique patterns that can be associated with specific diseases. These unique signatures have been used to identify specific disease states, such as cancer. One of the newer variants of SELDI is the matrix-assisted laser desorption/ionization–time-of-flight (MALDI-TOF) technique. This technique incorporates traditional SELDI technology with greater protein preparation, as the difference lies in the sample pretreatment. The protein is placed in a solid matrix instead of on a surface plate prior to ionization. This method makes it possible to more precisely control energy delivery. It also uses the characteristics of the matrix to enable better protein isolation through a high-throughput methodology that combines protein purification with precise protein identification.
Other common methods of protein identification are enzyme-linked immunosorbent assay (ELISA) and luminex. These techniques use immunoprecipitation to isolate and purify proteins from various sources. ELISA is an older technique and often requires large quantities of proteins. Each ELISA processing run requires recalibration and restandardization, which introduces variability. Furthermore, the volume of sample required is quite large and is typically an order of magnitude greater than that of other available technologies. Luminex uses newer technology and provides several benefits, including the ability to identify proteins from smaller samples, to run a larger number of samples within each batch and to reduce the variability between batches.
Protein microarrays are another method for protein isolation and identification. These techniques employ a high-throughput methodology analyzing hundreds to thousands of samples at once. Arrays with specific markers for proteins or protein moieties are employed in a chip format. This technology enables the identification of thousands of proteins in a single chip and requires only microliters of sample.
Ultimately any protein isolated in sufficient quantity can be identified through protein sequencing. All methods employed in protein sequencing require highly purified samples in relatively large quantities. This approach is reserved for proteins and polypeptides that have not yet been characterized or for which precise characterization is needed for drug development or biological understanding.
Biomarkers and mechanistic approaches to vasospasm
The search for biomarkers in SAH and vasospasm has been proceeding for decades. A remarkably large literature focused on the analysis of biomarkers in the context of human tissue and SAH has been developed. At different times various techniques and theoretical perspectives have been employed. Each approach has been based on a theoretical understanding as to the underlying mechanisms of vasospasm. Different approaches have evolved with developments in molecular biology. The first and perhaps most successful mechanistic approach emphasizes moderators of inflammation.
Inflammation
Inflammation has become a focus of research in vasospasm as many observations have confirmed this direct link. It has been demonstrated that leukocytosis and fever are associated with worse outcomes in SAH. Increased protein levels of several of the cellular adhesion molecules, including ICAM-1, VCAM-1, and the integrins, are associated with the development of vasospasm and worse clinical outcomes. Higher levels of these molecules have been measured in both the serum and CSF of patients who develop vasospasm. Other inflammatory markers, including the cytokine interleukin (IL) 2 (IL-2), IL-2–receptor antagonists, and soluble CD8 have been sampled in the CSF of patients with SAH and vasospasm and are elevated as compared to controls. However, they are not predictive of vasospasm. Furthermore, IL-6 and monocyte chemotactic protein–1 are higher in the CSF of patients who develop vasospasm. IL-8 and E-selectin are inflammatory markers that have been measured in the ventricular cisterns of patients with ruptured and nonruptured aneurysms. IL-6 levels are significantly elevated in the blood of patients with SAH in general. Complement has been measured using standard radioimmunoassays in the serum and CSF of patients with vasospasm. These studies have demonstrated increased levels of the C3 and C4a components of the complement cascade in the early stages of SAH. These levels decline after the first few days. Furthermore, CH50, C3, and C4 complement levels have been measured in serum of SAH patients and their levels have been predictive of vasospasm. After 5 to 10 days, however, serum complement levels were depleted in patients who went on to develop vasospasm. Other mediators of inflammation, such as arachidonic acid metabolites, have also been examined. To date, no study has documented an association with prostacycline or thromboxane in any serological compartment. However, 20-hydroxyeicosatetraenic acid, a metabolite of arachidonic acid, is elevated in the CSF of humans and animals with vasospasm. Chitotriosidase, a human chitinase member of family 18 glycosyl-hydrolases and selectively secreted by activated macrophages, has been measured in the CSF and serum of humans with SAH. Mean CSF chitotriosidase levels were found to be higher on days 5 and 7 of SAH. The serum levels were higher than controls at all times in SAH patients although no relationship between symptomatic vasospasm and outcome was observed.
Endothelial Activation
Endothelial activation is an important consideration in the setting of ischemic disease. Concentrations of soluble ICAM-1, soluble P-selectin, soluble E-selectin, and ED1-fibronectin are traditional markers of endothelial activation. Their levels have been sequentially measured throughout the course of treatment of patients with SAH. Concentrations of ICAM-1, soluble E-selectin, soluble P-selectin, ED1-fibronectin, von Willebrand Factor (vWf), and vWf propeptide have been measured in the serum of patients within 3 days of the onset of SAH to determine if any of the markers predict outcome. Early elevated vWf concentrations have been associated with poor outcome and the occurrence of ischemia. No other marker of endothelial activation has been associated with ischemia or poor outcome. This positive association of raised vWf concentrations with DINDs may reflect a predisposition to further ischemic injury via the formation of microthrombi in the cerebral circulation secondary to endothelial activation. Further studies are needed to confirm these findings and to better understand the molecular basis of this relationship.
Hemoglobin Degradation
Undoubtedly the breakdown products of red blood cells and hemoglobin induce vasospasm as it has been well demonstrated that blood volume plays a role in the risk of vasospasm in animal models and in human observation studies. It has also been shown that removal of the blood from the cisternal space facilitates a reduced risk of vasospasm. Deoxyhemoglobin is the most likely irritant triggering vasospasm. Oxyhemoglobin is the most vasoactive substance in subarachnoid blood and its concentration in CSF mirrors the time course of vasospasm. Free radicals, formed as a result of the toxic effects of subarachnoid blood and hemoglobin degradation, have been hypothesized to mediate many aspects of the pathophysiology of vasospasm. Emerging research implicates lipid peroxidation in addition to hemoglobin degradation byproducts in triggering vasospasm. The hydroxide generated in the process of lipid peroxidation acts as a vasoconstrictor and, furthermore, 15-hydroperoxyeicosatetraenoic acid (15-HPETE) and other lipid byproducts initiate vasospastic physiology. Their CSF levels have been associated with an increased risk of vasospasm. Measurements of cholesteryl ester hydroperoxides (CEOOH), which are generated during lipid peroxidation in plasma, may provide prognostic value. Plasma levels of CEOOH are elevated and peak 5 days after the onset of SAH. Furthermore, increased levels of CEOOH are associated with an increased mortality, are associated with an increased risk of vasospasm, and correlate with clinical outcome scales.
Calcium Metabolism
Vasospasm and vasoconstriction are abnormal prolonged contractions of the smooth muscles of the vasculature, resulting in the sustained narrowing of the artery. Calcium plays a central and integral role in this process. The contraction of the vessel walls involves the release of cytosolic calcium from a cellular reservoir, which leads to the contraction of smooth muscle in a sustained manner for prolonged periods. This can occur in a calcium dependent or independent manner. Many people have suggested that vasospasm stems from continuous elevation of cytosolic calcium, which causes sustained contraction of smooth muscle cells. CSF levels of intracellular species of proteins that bind calcium are lower in individuals with symptomatic vasospasm. Additionally, increased phosphorylation of key enzymes regulating cytosolic calcium reserves could also contribute to vasoconstriction in the setting of vasospasm. The roles of protein kinase C–, calponin-, and caldesmon-based mechanisms, as well as mechanisms mediated through mitogen-activated protein kinase, have been examined. To date, no proteomic approach has been applied to detect changes in the serum, CSF, or extracellular fluid level of any of these proteins. Levels of such proteins as calponin and caldesmon are potential markers of increased vasoconstriction mediating the prolonged contraction of smooth muscle. Phosphorylated-phosphorylated myosin light chain kinase is another potential protein marker for prolonged and sustained constriction of smooth muscle cells and merits further study.
Nitric Oxide
Endothelial relaxing factors, such as nitric oxide (NO) or NO-containing compounds, play a central role in the mediation of vasoconstriction in vasospasm. A variety of NO donors in humans and animals have demonstrated efficacy in the treatment of vasospasm and suggest that NO is a key player in vasoconstriction leading to clinical vasospasm. NO is a diffusible free radical gas with a half-life of seconds. To date, no efficient method exists for precisely detecting and measuring NO in vivo. The findings of several studies reporting temporal changes in CSF total nitrite/nitrate (NOx) levels after SAH vary considerably. Many have focused on detection of its metabolites, yet even these are difficult to measure because of their very short half-lives. The total nitrite/nitrate concentration has been determined by a vanadium-based assay with the colorimetric Griess reaction along with CSF oxyhemoglobin level as assessed by spectrophotometry. After an initial peak within the first 24 hours after SAH, CSF NOx decreases gradually and there is a significant correlation between CSF concentrations of NOx and levels of oxyhemoglobin. It has been demonstrated that patients with very good outcome have significantly lower CSF NOx than those with a worse outcome.
Endothelin
Endothelin, a 21–amino acid polypeptide, is a potent vasoconstrictor and has been implicated in the pathophysiology of vasospasm. Endothelin 1 has been found to be elevated in the CSF of patients with vasospasm. Two clinical trials have suggested efficacy for endothelin antagonists in the reduction of the observed vasoconstriction seen on angiograms. However, there was no significant reduction of DINDs. Endothelin has been measured in the CSF and serum using a variety of methods and its level correlates closely with the time course of vasospasm in virtually all mediums measured. Endothelin acts as the counterbalance to NO. However, its effects are long-acting than those of NO. Increases in endothelin’s efficacy would have a profound effect on the duration and degree of vasoconstriction. Evidence related to endothelin could indicate that the transition of the artery of normal tone to its constricted state stems from a disruption of its normal physiologic state. A promising goal for the treatment of cerebral vasospasm would be to determine effective measures to regulate these two systems. Further basic and translational research is needed.
The Coagulation Cascade
SAH is associated with a consumptive coagulopathy. D-dimers and other fibrin split products are elevated in the serum and CSF of patients with SAH. Fibrin, fibrin degradation products, fibrinopeptide A (FPA), and other coagulant by-products have been measured in the CSF of affected patients and these proteins act as markers of activation of the coagulation cascade. FPA has been selectively studied in patients with SAH with an initial elevation on days 0 to 1. However, this is followed by a subsequent decline over the ensuing days. The mean level of FPA is higher in patients with higher Fischer grade SAH and this corresponds to the risk of vasospasm. Furthermore, a significant increase in the FPA level of patients with infarction has been observed. Patients with decreased blood clearance on computed tomography scan demonstrate a significantly higher rate of infarction and a higher level of FPA. These results suggest that the thrombin activity present in CSF correlates with increasing Fischer grade, faster clearance of blood on computed tomography scan, and the development of vasospasm.
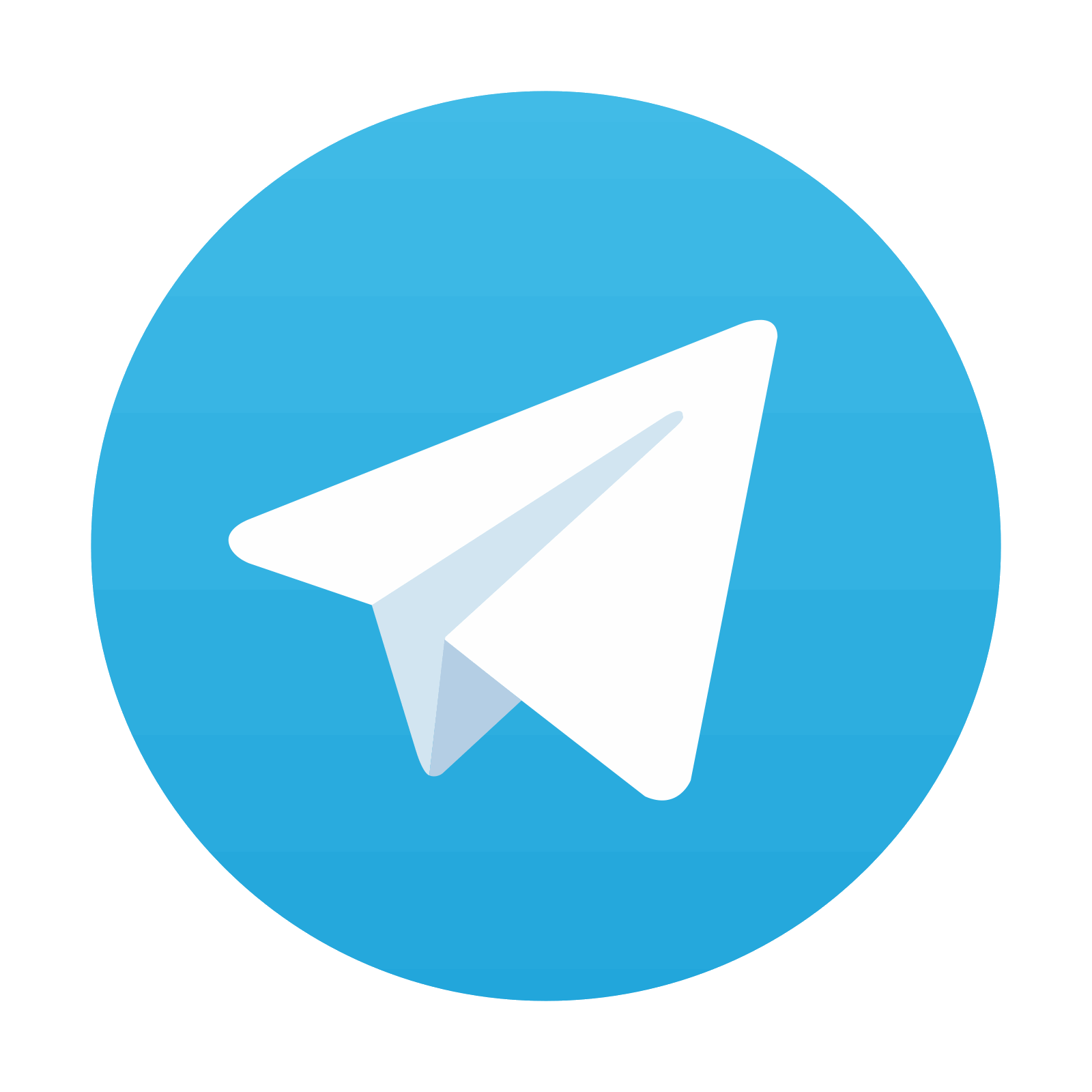
Stay updated, free articles. Join our Telegram channel
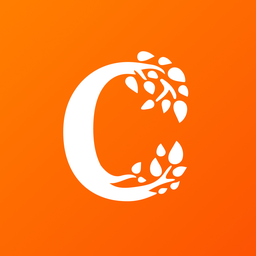
Full access? Get Clinical Tree
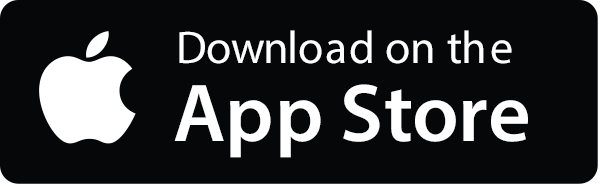
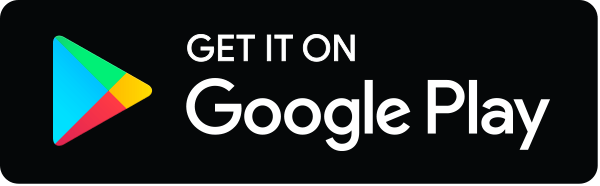