Biomechanics of Nonacute Cervical Spine Trauma
Parmenion P. Tsitsopoulos
Alexander J. Ghanayem
The cervical spine is subject to injury from various pathologic conditions other than acute trauma. Degenerative changes, inflammatory disorders, tumors, and iatrogenic injury can be the cause of neck pain, deformity, instability, and, finally, neurologic compromise. Each of these disorders affects the biomechanics of the cervical spine in a different way.
Degenerative changes of the cervical spine are anatomic adaptations to the continuous influence of wear and tear on the involved structures. Stability is achieved by osteophytic formation and disk height reduction in exchange of mobility and space available for the neural structures. Inflammatory disorders are mediated by a chronic systemic autoimmune response that results in characteristic anatomic changes throughout the body. Rheumatoid arthritis is the most common inflammatory disorder that affects the cervical spine. The inflamed synovium and the release of osteolytic factors alter cervical spine’s mechanical properties and lead to instability. Tumors of the cervical spine may be benign or malignant, primary or metastatic. Spine function can be affected by mass effect or bone destruction. An important aspect of tumor disease in spine biomechanics is the anticipated destabilization occasionally required for partial or complete tumor resection or the mechanical effects of medical treatment such as radiation therapy. Deformity results from the inability of the spine to maintain alignment under the complex stresses and loads associated with activities of daily living. Noticeably, stability depends on the nature of the bone, ligaments, muscles, and disks that resist these loads.
Depending on the underlying disease, surgery for nonacute cervical spine injuries has two main goals: decompression and stabilization. Because the effects of chronic pathology of the cervical spine can be related to the alteration of the mechanical properties of the tissues, this chapter addresses the biomechanics of nonacute cervical spine injuries and spinal cord impingement and the role and limitations of surgical treatment options.
INSTABILITY AND NONACUTE TRAUMA
Chronic disorders of the cervical spine can affect both mobility and stability. Information about normal cervical kinematics originates from both in vivo and in vitro studies. Kinematic data from in vivo studies have special clinical interest because they can help distinguish between healthy individuals and patients with cervical disorders (1,2). However, extrapolation of in vivo knowledge to in vitro experiments has often been difficult because of problems such as unknown moments and different definitions of neutral posture by different authors (3). On the contrary, in vitro data have been used to define the normal range of motion (ROM) in the various planes and thus to establish clinical criteria of instability (4). Using pure moments of 1.0 N m, Panjabi et al. (3) found that the greatest degree of flexion (12.3 degrees) occurred at C1-C2, whereas the greatest degree of extension (20.2 degrees) was observed at C0-C1. With axial moment loading, the greatest rotation (56.7 degrees) was seen at C1-C2. With lateral bending moments, the average ROM was 7.9 degrees, with no significant difference between segments (3).
Acute gross instability of the cervical spine can be easily recognized in static radiographs (5). Chronic instability can be detected on dynamic radiographs using data from in vivo and in vitro kinematic studies as a reference. The upper cervical spine is highly constrained by bony morphology and numerous ligamentous attachments, which make the establishment of radiologic criteria easier. Several measurements can be made from radiographs to document deformity and instability of the upper cervical spine and to plan treatment. The anterior atlantodental interval should be less than 3 mm. Superior migration of the dens can be assessed with McGregor’s line. A migration of the tip of the dens of more than 4.5 mm is indicative of vertical settling of the occiput (6). In dens fractures, initial displacement of greater than 5 mm is associated with high nonunion rates and is an indication for surgical stabilization (7).
In the lower cervical spine, the viscoelastic intervertebral disk and the coupled kinematics make recognition of clinical instability and the establishment of specific instability criteria more difficult. Overall, a functional spinal unit (FSU) that exhibits more than 20 degrees of sagittal rotation in dynamic flexion-extension radiographs has been found to be above all normal limits in both in vivo (8,9) and in vitro studies (3,10) and is considered unstable. Sagittal mobility of an FSU of more than 11 degrees compared with the presumed intact segment above or below is also indicative of instability (4).
Translations, as a component of normal coupling of cervical spine kinematics, occur mainly at the C0-C1 and C1-C2 level and have a dorsal direction, with an average value of 11.4 mm (3). This value is well inside the tolerance of the spinal canal space available for the cord (SAC) in the upper cervical spine. In contrast, anterior-posterior translations are minimal in the lower cervical spine (average 1.2 mm) (3). Considering 2.7 mm as the highest reported value of anterior-posterior translation in vitro (3,10,11) and an average radiographic magnification to be 30%, Panjabi et al. (4) proposed the criterion of 3.5 mm of maximum normal sagittal plane translation.
Defining instability in cases of degenerative painful cervical syndromes is more difficult. In most cases, a degenerative segment has either normal or decreased ROM. The stabilizing effect of neck muscles is difficult to be determined from in vitro data. Patients with neck pain may have decreased isometric muscle strength (12,13). Interestingly, women have about half of the neck strength of men and experience neck pain more frequently (14). Physiotherapy with conditioning of the neck musculature has been shown to improve the symptoms of degenerative disease of the spine.
Clinical studies have shown that temporary external stabilization of a suspected painful and unstable segment can predict the pain relief expected by an arthrodesis with good accuracy (15). Panjabi (16) has introduced the term neutral zone (NZ), describing it as the part of the ROM of a spinal segment at which minimal force application results in large displacement (laxity). The effects of external fixation on the load-deformation curve of the stabilized segment have also been measured in vitro. It was found that the greatest decrease occurred in the NZ (69%), with the total ROM losing only 39% of the intact value (15). These findings led Panjabi to redefine clinical instability as follows: “Clinical instability is a significant decrease in the capacity of the stabilizing system of the spine to maintain the intervertebral NZs within the physiologic limits, so that there is no neurologic dysfunction, no major deformity, and no incapacitating pain.” (4). The NZ is larger in segments with the greatest ROM. The larger NZ for flexion occurred in C1-C2 (4.6 degrees), for extension in C0-C1 (13.9 degrees), for axial rotation at C1-C2 (39.6 degrees), and for lateral bending at C3-C4 and C4-C5 (4.4 degrees at each level) (3).
DEFORMITY AND NONACUTE TRAUMA
The final result of nonacute instability is cervical deformity. Deformity may produce spinal cord or nerve compression and pain. The most frequent cervical deformity is kyphosis. The most frequent type is iatrogenic, which results from excessive removal of posterior elements (17,18). Pal and Sherk (19) demonstrated that in the cervical spine, 36% of the load is transmitted through the bodies and disks and 64% through the facet joints. The lamina forms a closed ring that increases the stiffness of the articular processes. Disruption of the integrity of this dorsal arch facet complex can cause instability (20,21), shifting the weight-bearing axis ventrally. The resultant kyphosis requires constant muscular contraction to maintain upright head posture. Eventually, fatigue and pain occur, the deformity progresses, and increased loads are applied on the vertebral bodies and disks resulting in accelerated degeneration and formation of ventral osteophytes. The spinal cord is then compressed ventrally on the osteophytes formed in the apex of the kyphotic deformity. The final stage of this process is the evolution of myelopathy. A poorly planned surgical decompression may also aggravate the kyphotic deformity. It is clear that any preexisting kyphosis significantly increases the risk of deformity after cervical laminectomy (22, 23 and 24). Kyphotic deformity after dorsal surgery is more pronounced in children (25). Increased load shifting from the posterior elements to the
anterior column will compress the cartilaginous end plates and will result in wedging of the vertebral bodies (26).
anterior column will compress the cartilaginous end plates and will result in wedging of the vertebral bodies (26).
![]() Figure 6.1. Lateral radiograph of a patient with longstanding rheumatoid arthritis. This demonstrates diffuse instability with multiple levels of spondylolisthesis (stair-step spine). |
Another frequent cause of cervical spine deformity is the chronic instability produced by the erosive process of inflammatory disorders (Fig. 6.1). A marked deformity of the upper cervical spine is the anterior atlantoaxial subluxation that is present in 45% of patients with rheumatoid arthritis (27, 28, 29 and 30). The transverse ligament of the atlas has histologic features similar to the articular cartilage and, as such, is affected early in the development of rheumatoid disease (31). The amount of fibrocartilage present at the level of the atlantodental articulation suggests that the ligament is always under preload and thus is an important stabilizer of the upper cervical spine (31). A finite-element study of the craniovertebral junction found that a 75% reduction in the stiffness of the transverse ligament results in atlantoaxial subluxation. Subsequent simulated involvement of the alar ligaments and capsules produced advanced atlantoaxial subluxation and severe deformity (32).
Tumor involvement of the cervical spine (either primary or metastatic) will also produce instability, deformity, and neurologic injury. En bloc excision of a tumor as described by Enneking (33) is only rarely possible in the cervical spine due to the small size of the vertebrae, the neurovascular tissues that are in the proximity, and the need for combined ventral and dorsal approaches. However, when possible, it can provide good disease-free survival rates (34) An intralesional or marginal excision is the only option in most cases with the margins of excision and tumor aggressiveness being strong predictors of outcome (35).
BIOMECHANICS OF NONACUTE NEUROLOGIC TRAUMA
Contrary to the volume of information available for the biomechanics of the osseoligamentous structures of the spine, few data exist on the physical properties of the spinal cord and the spinal nerves. The spinal cord together with the vascular pia has an elastic strain value of about 10% the original length. This deformation is possible with minimal stress (0.01 N). Further strain is opposed with highly increased stress. Failure stress was found to be about 20 to 30 N (4). The deforming forces acting on the cord produce a variety of compressive, tensile, and shear forces. A bending moment will create tensile stresses on the convex side of the bent spinal cord and compressive forces on the concave side. These forces may produce ischemia, cell injury, and finally permanent neural degeneration. Spinal cord compression may be static or dynamic, but usually both mechanisms coexist, producing myelopathy.
Static compression is the result of gradual reduction of spinal canal size, which reduces the SAC. The effect of static cord compression has been well documented by Ogino et al. (36) in a clinicopathologic study where myelopathic patients were followed clinically until death, and in autopsy, the pathologic changes of the cord were investigated. The extent of spinal cord compression correlated well with the severity of pathologic changes in the cord as well as with the clinical symptoms.
The midsagittal bony diameter from C3 to C7 in whites has been reported to be on average between 14.2 mm (37) and 17 mm (38,39). The semicompressive posterior longitudinal ligament (average width 3.5 mm) and the elastic ligamentum flavum (average width 2.3 mm) normally reduce the effective average canal diameter to 12.7 mm. There are slight variations between sexes and more significant variations between races, with Asians having reportedly smaller dimensions (40). The spinal cord measures approximately 10 mm in diameter (range, 8.5 to 11.5 mm) (41). Several authors have reported on the minimum spinal canal diameter that can be tolerated by the spinal cord. An anterior-posterior diameter of less than 12 mm will produce cord compression and symptoms (42,43). Myelopathic changes are more likely to develop in a congenitally narrowed cervical canal, where additional narrowing by spondylotic changes results in spinal cord compression (44). Pavlov’s ratio (spinal canal width to vertical body width) should be 1. A ratio less than 0.8 is indicative of spinal stenosis, and an increased risk for cord injury exists (45). In contrast with the lower cervical spine, the upper cervical spine normally has ample SAC. This explains the high tolerance of displacement accepted by the cord in dens fractures and inflammation-induced subluxations.
Dynamic injury to the cord during normal cervical spine movements is the result of direct impact or ischemia produced by the occlusion of intraspinal vessels as the cord buckles around osteophytes or other canal-occupying lesions. Changes of the transverse dimensions of the normal spinal canal are minimal during the physiologic ROM. The length of the spinal canal increases with flexion and decreases with extension, imposing a spectrum of compressive, tensile, and shear stresses on the spinal cord during normal kinematics (Fig. 6.2). A bending moment creates tensile stress on the convex side of the spinal cord and compressive stress on the concave side. The normal spinal cord tolerates these physiologic stresses well. In contrast, when the mechanical properties of the cervical spine are altered, the cord experiences significant deformations. In degenerative spine disease in particular, the ligamentum flavum loses its normal elasticity. As the neck extends, the ligamentum flavum buckles inward (1,8,39). This results in significant decrease of the spinal canal cross-sectional area. In addition, the spinal cord shortens in extension and its cross-sectional area increases (46). The combination of reduced transverse canal dimensions with increased transverse spinal cord dimensions increases the risk for myelopathy from direct repetitive trauma.
Ischemia is another important factor in the development of nonacute spinal cord injury (47). Breig et al. (46) have supported the hypothesis that deformation of the cord in the transverse plane produces selective vessel occlusion as a result of the cord’s vascular anatomy. The spinal cord vessels are normally able to compensate for deformations during physiologic kinematics. The subarachnoid longitudinal arteries supplying the dorsal spinal cord run on the dorsal surface on a zigzag course, so they can compensate for the elongation that occurs during cervical spine flexion or ventral compression. The arteries supplying the anterior columns run in an anterior-posterior direction, so they relax under ventral spinal cord compression and circulation compromised. In contrast, the transverse penetrating vessels arising from the anterior sulcal
artery are elongated and occluded as the cord flattens and stretches in the lateral dimension under anteriorposterior compression. The resulting ischemia produced by this mechanism affects mainly the medial aspect of the dorsal columns and the lateral corticospinal tracts. Of note, tethering from the dentate ligaments and the cervical roots may further affect cord’s vascularity.
artery are elongated and occluded as the cord flattens and stretches in the lateral dimension under anteriorposterior compression. The resulting ischemia produced by this mechanism affects mainly the medial aspect of the dorsal columns and the lateral corticospinal tracts. Of note, tethering from the dentate ligaments and the cervical roots may further affect cord’s vascularity.
Nerve root injury occurs mostly around the intervertebral foramina and can be static or dynamic. Foraminal stenosis may be the consequence of an intervertebral disk herniation (resulting in disk collapse and dorsolateral protrusion) (48,49), hypertrophy of the facet joints (50), and/or the uncinate processes (49). The dimensions of the neural foramina are significantly reduced during extension and ipsilateral bending of the cervical spine and are increased during flexion and contralateral bending (51,52). The Spurling maneuver is an example of a clinical provocative maneuver that reproduces the symptoms of an irritated root by reducing the size of the foramen. The beneficial effect of therapeutic traction under neck flexion is also easily explained in a similar manner (53).
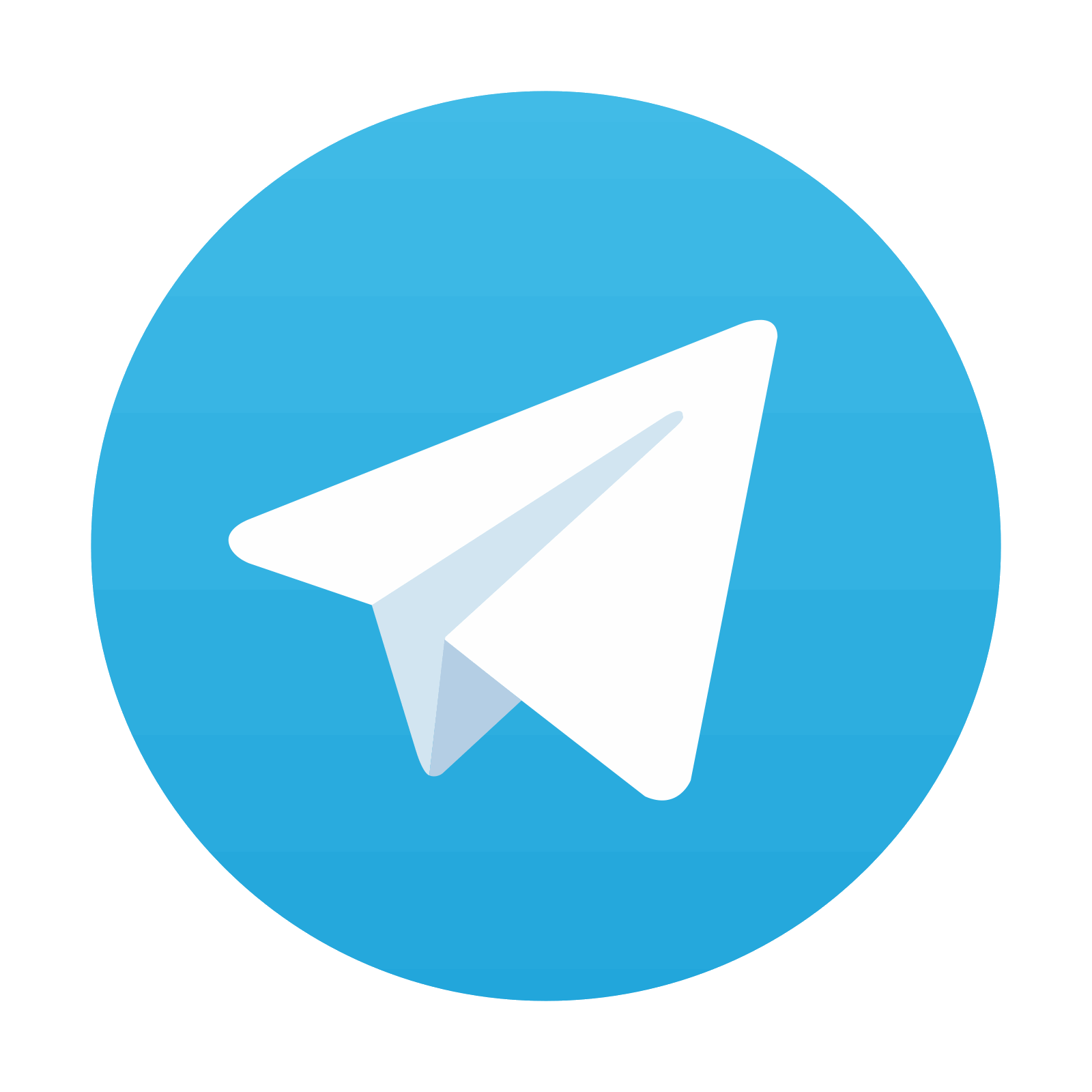
Stay updated, free articles. Join our Telegram channel
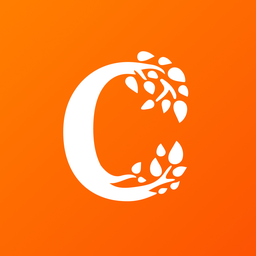
Full access? Get Clinical Tree
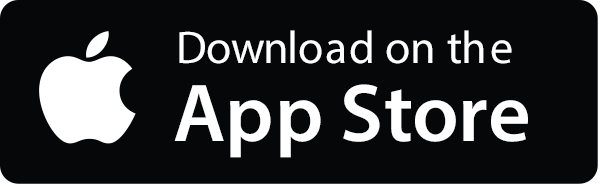
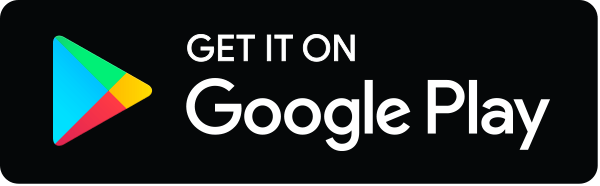