Abstract
Avoiding secondary brain injury due to hypoxia and/or ischemia is the mainstay and declared goal of neurocritical care. Monitoring the brain’s overall condition after trauma with intracranial pressure and cerebral perfusion pressure alone may be insufficient to prevent secondary brain damage. Therefore, additional parameters to quantify brain tissue oxygenation have been developed and introduced into clinical practice. In this chapter, reflectance spectrophotometry, near-infrared reflectance spectroscopy, oxygen electrode, optode, and briefly, imaging studies are presented. The technical details of each method are presented, and the evidence regarding their clinical usefulness to reduce secondary brain damage is discussed.
Keywords
Brain tissue oxygenation, Intracranial bleeding, Neurocritical care, NIRS, Optode, Oxygen electrode, Reflectance spectrophotometry, Secondary brain injury, Traumatic brain injury
Contents
Introduction 250
Basic Principles 251
Reflectance Spectrophotometry 254
Near-Infrared Reflectance Spectroscopy 254
Oxygen Electrode 255
Optode 256
Other Methods 256
Understanding the Equipment 256
Reflectance Spectrophotometry 256
Near-Infrared Reflectance Spectroscopy 257
Oxygen Electrode 259
Optode 260
Indications and Contraindications 263
Reflectance Spectrophotometry 263
Near-Infrared Reflectance Spectroscopy 263
Oxygen Electrode/Optode 264
Readings and Interpretation 264
Reflectance Spectrophotometry 264
Near-Infrared Reflectance Spectroscopy 265
Oxygen Electrode 265
Optode 266
Effect of Anesthetics 266
Reflectance Spectrophotometry 266
Near-Infrared Reflectance Spectroscopy 267
Oxygen Electrode/Optode 267
Advantages and Disadvantages 268
Reflectance Spectrophotometry 268
Near-Infrared Reflectance Spectroscopy 268
Oxygen Electrode/Optode 268
Current Evidence 271
SjvO 2 and CPP 271
SjvO 2 Versus PET 271
SjvO 2 in Various Conditions 271
NIRS in Various Conditions 272
Oxygen Electrode Versus Optode 273
PbtO 2 Versus SjvO 2 273
PtbO 2 in Various Conditions 274
Current Evidence for TBI 274
Current Evidence for Vasospasms in SAH 275
Current Evidence for Brain Ischemia 275
Summary of Current Evidence 275
Suggested Readings 277
References 277
Introduction
Primary brain injury results from traumatic or disease-related impact. Two major problems are associated with these injuries. First, damage after primary brain injury cannot be undone even with the best possible management. Second, secondary brain injury is inherent due to a variety of cellular processes. Hence, avoiding secondary brain injury due to hypoxia and/or ischemia is the mainstay and declared goal of neurocritical care.
Historically, monitoring of the brain’s overall condition after injury began with intracranial pressure (ICP) and cerebral perfusion pressure (CPP) monitoring and was based on the Monro-Kellie doctrine, described more than two centuries ago. Further investigations originated from the assumption that ICP and CPP monitoring alone are not enough to avoid secondary brain injury, as these parameters do not sufficiently reflect cerebral blood flow (CBF) and oxygenation in the injured and the intact parts of the brain. Even when measuring a normal ICP and CPP, one cannot assume that there is uninterrupted oxygen and glucose delivery to the region of primary insult and particularly to at-risk regions in close proximity. In 1993, monitoring of partial pressure of brain tissue oxygen (PbtO 2 ) was introduced to further improve outcome in brain-injured patients by prevention of hypoxic events. Brain cortex PO 2 was measured after craniotomy, and low tissue PO 2 levels were found to be associated with ischemia. PbtO 2 measurements are used to quantify brain tissue oxygen delivery. PbtO 2 represents PO 2 in a small catchment area of tissue below the probe and is influenced by the O 2 content of the vessels in the investigated area. It is important to note that there is no perceived difference between capillary vessels and tissue PbtO 2 . PbtO 2 reflects oxygen availability for aerobic energy production and represents the balance of O 2 delivery and consumption, both of which are influenced by alterations in the microcirculation. Over time, various methods for assessment of brain tissue oxygenation have been developed and investigated not exclusively under pathological conditions. Table 9.1 gives an overview on the currently available and commonly used measurement methods for brain tissue oxygenation.
Technique | Device/measurement: variable | Penetration depth |
---|---|---|
Reflectance spectrophotometry | Hemoglobin oxygen saturation (e.g., jugular venous oxygen saturation: SjvO 2 ) | 250 μm |
Near-infrared reflectance spectrometry (NIRS) | Various commercial devices based on discriminative NIRS methodology : cerebral blood volume, [Hb]/[O 2 Hb], cytochrome aa 3 | Depends on NIRS methodology |
Clark electrode | Oxygen electrode: PO 2 | Implantable electrodes: 15–20 μm Surface devices: 20 μm |
Luminescence: fluorescence | Optode: PO 2 , PCO 2 , pH | Unknown |
Basic Principles
Methods of brain tissue oxygenation measurement differ in spatial resolution ( Table 9.1 ). In this chapter, the methods will be discussed beginning with focal or regional monitoring followed by monitoring of global brain tissue oxygenation. They can be summarized as follows:
- •
Clinically used methods:
- •
Reflectance spectrophotometry (e.g., in jugular venous oxygen saturation (SjvO 2 ))
- •
Near-infrared reflectance spectroscopy (NIRS)
- •
Oxygen electrode (e.g., in PbtO 2 )
- •
Optode (e.g., in PbtO 2 )
- •
Other methods:
- –
Positron emission tomography (PET)
- –
Magnetic resonance spectroscopy (MRS)
- –
Magnetic resonance imaging (MRI)
- –
- •
Worth noting, the variables discussed in the literature and everyday practice are not always easily assigned to a particular method. Table 9.2 reviews the variables that are most frequently used.
Variable attributed to this device | General term |
---|---|
Reflectance spectrophotometry
| Global cerebro-venous Hb oxygen saturation |
NIRS a , : SctO 2 equivalents
| [Hb]/[O 2 Hb] |
Oxygen electrode:
| PbtO 2 b |
b Brain tissue oxygen partial pressure; synonym: PbO 2 ; PtO 2 refers to tissue oxygenation in general (i.e., not specific for the brain).
All methods explained here are not exclusively valid for brain monitoring ( Table 9.3 ), and some general advantages or disadvantages should be considered when choosing one method over another ( Table 9.4 ). Invasiveness was suggested to be relevant for the development of a local or generalized infection. Current sparse data reveal that infections related to probe implantation rarely develop. Further, there are only very few reported cases of relevant bleeding post insertion of intraparenchymal probes.
Method | Indications |
---|---|
Reflectance spectrophotometry |
|
NIRS |
|
Oxygen electrode |
|
Optode |
|
Context of use | Invasiveness | Ease of use | Reliability | Safety | |
---|---|---|---|---|---|
Reflectance spectrophotometry | Clinical | ± | Yes | Medium | High |
NIRS | Clinical | − | Yes | Medium | High |
Oxygen electrode | Clinical | + | No | High | Good |
Optode | Clinical | + | Yes | High | Good |
If a device is easy to use or a method easy to establish, there is lower risk for incorrect results. Such devices or methods can be more easily implemented in clinical studies and treatment algorithms. The reliability of various methods to monitor brain tissue oxygenation is reviewed in the Current Evidence section.
Reflectance Spectrophotometry
Spectrophotometry in this context quantifies reflection or transmission properties of tissue depending on the wavelength: light is emitted and then absorbed, reflected, or transmitted by molecules such as O 2 as it passes through tissue. As hemoglobin saturation status alters proportional to the amount of bound oxygen, it changes in color, hence light absorption. Light passes more easily in blood with high O 2 content.
A spectrometer produces, disperses, and measures light, whereas a photometer measures the intensity of light. In reflectance photometry, the reflected light (i.e., illumination) is measured after diffused light provokes a reaction in a medium.
Reflectance spectrophotometry measures the intensity of the reflected light compared to the light intensity of a reference. As O 2 concentration increases, light absorption increases linearly while light transmission decreases exponentially.
Near-Infrared Reflectance Spectroscopy
The basic principle of NIRS is based on the transmission and absorption of near-infrared (NIR) light in the tissues of interest. Light generated at specific wavelengths is then detected after passing through and reflected by tissues (e.g., brain, Fig. 9.1 ). The NIR spectrum of commercial devices for clinical use ranges from 700 to 950 nm and is suitable for cerebral tissue oxygen saturation (SctO 2 ) for two reasons. First, the relative transparency of tissue to light in this wavelength range allows transition of biological tissue permitting measurement of absorption peaks of biological molecules in this region of the NIR spectrum. Second, several biological molecules (chromophores; see explanation subsequently) happen to reveal distinct NIR spectra.

There are several NIRS techniques, and all are attributed to the measurement of optical attenuation (i.e., the total loss of light due to absorption and scattering). The attenuation of light at a specific wavelength is described by the Beer–Lambert law. It states that attenuation is directly proportional to the following three variables:
- •
chromophore concentration
- •
distance between source and detector traveled by light
- •
absorption coefficient of the chromophore (i.e., the tissue)
NIRS measures the concentration of three chromophores: oxyhemoglobin (O 2 Hb), deoxyhemoglobin (HHb), and cytochrome aa 3 . The first two allow measurement of Hb tot , HbO 2 , and cerebral blood volume.
Ideally, the only cause for attenuation between the light emitting source and the detector is light absorption by chromophores. However, biological tissue presents a more complex situation, beyond the scope of this overview. The different commercially available devices include special algorithms to compensate for the effect of the nonchromophore tissue.
In the end, output values from both reflectance spectrophotometry and NIRS can be derived in a similar way. They follow an algorithm based on measured changes of light transmission that are converted into a physiological signal (see the Readings and Interpretation section).
Oxygen Electrode
The oxygen electrode derives from the classic Clark electrode, and it measures the voltage change, which is proportional to the amount of O 2 molecules between the reference electrode (anode) and the measuring electrode (cathode) where they are reduced.
Oxygen electrodes can be implemented to various systems, involving the application of probes to the surface or their introduction into a liquid or gaseous medium of interest.
Optode
Optodes are optical sensors that can be used to measure the oxygen saturation. Changes in phosphorescence quenching (i.e., attenuation of fluorescence) being measured by the optode depend on the amount of O 2 . The dye responsible for the O 2 measurement changes its color depending on the amount of O 2 and is located within the electrode but not in the tissue itself (see the Understanding the Equipment section).
In addition to PO 2 , variations of PCO 2 and hydrogen ion concentrations lead to modification of fluorescence and absorbance of the indicators. Therefore, PO 2 , PCO 2 , and pH can be measured using this method.
Other Methods
Using imaging techniques bears the advantage that the metabolic parameters in a specific area of interest can be investigated and compared to other areas. However, imaging provides only a “snap shot” and cannot be classified as monitoring in the classical sense. Nevertheless, these methods are noninvasive, providing a safe method for the assessment of cerebral O 2 metabolism.
Positron Emission Tomography
PET can be used to calculate regional cerebral blood volume, O 2 metabolism, blood flow, and O 2 extraction fraction and has been found to be a highly reliable tool. However, due to the complexity of this method, it remains a research tool rather than a clinical monitor.
Magnetic Resonance Spectroscopy and Magnetic Resonance Imaging
Magnetic resonance methods can also be used to assess oxygenation of a certain area of interest and have been used to measure tumor oxygenation. Under various oxygen conditions, these methods have been used as a marker of tissue pO 2 , as dissolved molecular oxygen serves as an endogenous contrast agent.
Understanding the Equipment
Reflectance Spectrophotometry
This method allows for a continuous estimation of oxygen saturation of arterial or venous hemoglobin. In the context of brain injury, probes are typically inserted into the jugular bulb to measure cerebro-venous oxygen saturation.
Jugular bulb saturation (SjO 2 ), or the arterio-jugular oxygen content difference (AJDO 2 ), is calculated as the difference between the arterial and jugular oxygen content in paired blood samples. It provides information about the adequacy of global CBF in relation to metabolic demands but is only correct if the cerebral metabolic rate for oxygen (CMRO 2 ) does not change independently of CBF. Therefore, coupling between flow and metabolism must be intact. The placement of catheters in the jugular bulb allows sampling of blood that drains almost exclusively from the intracranial circulation. Despite the fact that blood is usually sampled from only one jugular bulb, it is assumed that the values relate to global CBF rather than hemispherical CBF. However, typically only two-thirds of the sampled blood is drained from the ipsilateral hemisphere. In addition, interindividual variability of venous drainage of the brain is large, explaining why methods relying on blood sampling from just one of the jugular bulbs are prone to the influence of asymmetry of cerebral venous drainage. It is impossible to predict which side in a specific patient will provide more important data, and there is no consensus on which side should be cannulated. Generally, the right internal jugular vein is preferred because it is often the dominant vessel. Alternatively, the side with the larger jugular foramen can be used or the side on which a compression of the jugular vein causes a greater increase in ICP. The catheter tip should lie at the level of the first or second cervical vertebral body ( Fig. 9.2 ), which is above the point at which the jugular vein receives its first extracranial tributary, the facial vein. Extracranial contamination at this level is considered to be about 3%. Correct position of the catheter should be confirmed with a lateral cervical spine X-ray. Blood can be sampled intermittently, or a fiberoptic catheter can be used to continuously determine SjO 2 . If samples are withdrawn too quickly, falsely elevated values may be found because of retrograde aspiration of extracranial blood. A rate of blood withdrawal of less than 2 mL/min should be used. Fiberoptic catheters need to be recalibrated at regular intervals and are susceptible to artifacts as a result of the catheter position inside the vessel. It has been shown that when a fiberoptic catheter is used for continuous SjO 2 monitoring, “time of good quality data” can be as low as 43% of the monitoring time.

Near-Infrared Reflectance Spectroscopy
In this noninvasive method, NIRS devices are attached to the region of interest, usually the forehead.
NIRS values may be influenced by factors such as ambient light, skin pigmentation, temperature, or even variable location of the intracranial sinuses. Furthermore, vasopressors have been shown to have an influence. Devices typically display tissue oxygenation index (TOI) or regional tissue O 2 saturation (rSO 2 ). The precise volume and location of the monitored tissue remains unknown.
The display of the INVOS ® monitor ( Fig. 9.3 ), for example, is split into four panels. Two separate trend monitors can be found on the left side, one each for the left and right frontal sample volume. On the right side, the updated rSO 2 value is shown numerically and compared to the baseline value, which is indicated separately below the updated value. A fixed horizontal on the trend monitor (red line) indicates the threshold rSO 2 value below which the patient should not fall. Also below the updated rSO 2 value (red line), the variable (min%) of the area under the curve indicates both the magnitude of low rSO 2 values (%) and time spent below the threshold rSO 2 value (min).

Oxygen Electrode
Oxygen electrodes for the measurement in tissue and microcirculation consist of silver-, gold-, or platinum-reducing oxygen due to a negative polarizing voltage. The change in voltage between the reference electrode (anode) and the measuring electrode (cathode) is proportional to the amount of oxygen molecules that are reduced at the cathode. In the Clark electrode, both anode and cathode are placed behind an oxygen-permeable and electrically insulating membrane ( Fig. 9.4 ). Transport of oxygen occurs due to diffusion from the surrounding tissue with a high PO 2 , to the area behind the membrane of the electrode where the oxygen pressure is close to zero. To obtain reliable measurement, the O 2 consumption of the electrode should be small compared to the local O 2 level within the tissue; otherwise the electrodes have the potential to alter their own environment. The sensitivity of the electrode is determined by the diffusion constant of the electrode membrane, while the surface of the electrode defines the amount of oxygen generating the current after combining the hydrogen. Calibration of these oxygen electrodes in a solution of known oxygen tension is needed prior to each measurement.
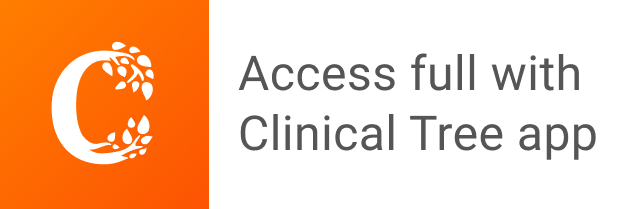