Cellular Mechanisms of Implicit Memory Storage and the Biological Basis of Individuality
Storage of Implicit Memory Involves Changes in the Effectiveness of Synaptic Transmission
Habituation Results from an Activity-Dependent Presynaptic Depression of Synaptic Transmission
Sensitization Involves Presynaptic Facilitation of Synaptic Transmission
Classical Fear Conditioning in Flies Uses the cAMP-PKA-CREB Pathway
Memory for Learned Fear in Mammals Involves the Amygdala
Habit Learning and Memory Require the Striatum
THROUGHOUT THIS BOOK WE HAVE EMPHASIZED that all behavior is a function of the brain and that malfunctions of the brain produce characteristic disturbances of behavior. Behavior is also shaped by experience. How does experience act on the neural circuits of the brain to change behavior? How is new information acquired by the brain and, once acquired, how is it remembered?
In the previous chapter we saw that memory is not a single process but has at least two major forms. Implicit memory operates unconsciously and automatically, as in the memory for habits and perceptual and motor skills, whereas explicit memory operates consciously, as in the memory for people, places, and objects. Long-term storage of explicit memory begins in the hippocampus and the medial temporal lobe of the neocortex, whereas long-term storage of implicit memory requires a family of structures: the neocortex for priming, the striatum for skills and habits, the amygdala for learned fear, the cerebellum for learned motor skills, and certain reflex pathways for nonassociative learning such as habituation and sensitization (Figure 66–1).
Figure 66–1 Two forms of long-term memory involve different brain systems. Implicit memory involves the neocortex, striatum, amygdala, cerebellum, and in the simplest cases the reflex pathways themselves. Explicit memory requires the medial temporal lobe and the hippocampus, as well as certain areas of neocortex (not shown).
Over time, explicit memories are transferred to different regions of the neocortex. In addition, many cognitive, motor, and perceptual skills that we initially store in explicit memory ultimately become so ingrained with practice that they become stored as implicit memory.
The transference from explicit to implicit memory and the difference between them is dramatically demonstrated in the case of the English musician and conductor Clive Waring, who in 1985 sustained a viral infection of his brain (herpes encephalitis) that affected the hippocampus and temporal cortex. Waring was left with a devastating loss of memory for events or people he had encountered even a minute or two earlier. Yet he could still read music, perform on the piano, and conduct a chorale. Under these circumstances it was clear that many aspects of his basic personality—the biological basis of his individuality—were still intact. Once a performance was completed, however, he could not remember a thing about it.
Similarly, William deKooning, the abstract expressionist painter, developed Alzheimer disease and severe disturbances of explicit memory. As the disease progressed and his memory for people, places, and objects deteriorated, he nevertheless continued to produce important and interesting paintings. This aspect of his creative personality was relatively untouched.
In this chapter we examine the cellular and molecular mechanisms that underlie implicit memory in invertebrate and vertebrate animals. In the next chapter we examine the biology of explicit memory storage in mammals.
Storage of Implicit Memory Involves Changes in the Effectiveness of Synaptic Transmission
Studies of elementary forms of implicit learning—habituation, sensitization, and classical conditioning—provided the groundwork for understanding the neural mechanisms of memory storage. Such learning has been analyzed in simple invertebrates and in a variety of vertebrate reflexes, such as the flexion reflexes, fear responses, and the eye blink. These simple forms of implicit learning involve changes in the effectiveness of the synaptic pathways that mediate the behavior.
Habituation Results from an Activity-Dependent Presynaptic Depression of Synaptic Transmission
Habituation is the simplest form of implicit learning. It occurs, for example, when an animal learns to ignore a novel stimulus. An animal reacts to a new stimulus with a series of orienting responses. If the stimulus is neither beneficial nor harmful, the animal learns to ignore it after repeated exposure.
The physiological basis of habituation was first investigated by Charles Sherrington while studying posture and locomotion in the cat. Sherrington observed a decrease in the intensity of certain reflexes in response to repeated electrical stimulation of the motor pathways. He suggested that this decrease, which he called habituation, is caused by diminished synaptic effectiveness in the stimulated pathways. Habituation was later investigated at the cellular level by Alden Spencer and Richard Thompson. They found close cellular and behavioral parallels between habituation of a spinal flexion reflex in cats (the withdrawal of a limb from a noxious stimulus) and habituation of more complex human behaviors. They showed that during habituation the strength of the input from local excitatory interneurons onto motor neurons in the spinal cord decreased. Connections to interneurons from sensory neurons innervating the skin were unaffected.
Because the organization of interneurons in the vertebrate spinal cord is quite complex, it was difficult to analyze further the cellular mechanisms of habituation in the flexion reflex. Progress required a simpler system. The marine mollusk Aplysia californica, which has a simple nervous system of about 20,000 central neurons, proved to be an excellent system for studying implicit forms of memory.
Aplysia has a repertory of defensive reflexes for withdrawing its respiratory gill and siphon, a small fleshy spout above the gill used to expel seawater and waste (Figure 66–2A). These reflexes are similar to the withdrawal reflex of the leg studied by Spencer and Thompson. Mild touching of the siphon elicits reflex withdrawal of both the siphon and gill. With repeated stimulation these reflexes habituate. As we shall see, these responses can also be dishabituated, sensitized, and classically conditioned.
Figure 66–2 Short-term habituation of the gill-withdrawal reflex of the marine snail Aplysia.
A. A dorsal view of Aplysia illustrates the respiratory organ (gill) and the mantle shelf, which ends in the siphon, a fleshy spout used to expel seawater and waste. Touching the siphon elicits the gill-withdrawal reflex. Repeated stimulation leads to habituation.
B. This simplified circuit shows key elements of the gill-withdrawal reflex as well as sites involved in habituation. Approximately 24 mechanoreceptor neurons in the abdominal ganglion innervate the siphon skin. These sensory cells make excitatory synapses onto a cluster of six motor neurons that innervate the gill as well as on interneurons that modulate the firing of the motor neurons. (For simplicity only one of each type of neuron is illustrated here.) Touching the siphon leads to withdrawal of the gill (dashed outline shows original gill size; solid outline shows maximal withdrawal).
C. Repeated stimulation of the siphon sensory neuron (top traces) leads to a progressive depression of synaptic transmission between the sensory and motor neurons, seen as a decrease in size of the motor neuron EPSP with no change in the action potential (AP) in the presynaptic sensory neuron. In a separate experiment repeated stimulation of the siphon results in a decrease in gill withdrawal (habituation). One hour after repetitive stimulation both the EPSP and gill withdrawal have recovered. Habituation is now known to involve a decrease in transmitter release at many synaptic sites throughout the reflex circuit (part B). (Adapted, with permission, from Pinsker et al. 1970; Castellucci and Kandel 1974.)
The neural circuit mediating the gill-withdrawal reflex in Aplysia has been studied in detail. Touching the siphon excites a population of mechanoreceptor sensory neurons that innervate the siphon. The release of glutamate from sensory neuron terminals generates fast excitatory postsynaptic potentials (EPSPs) in interneurons and motor cells. The EPSPs from the sensory cells and interneurons summate on motor cells both temporally and spatially, causing them to discharge strongly, thereby producing vigorous withdrawal of the gill. If the stimulus is repeated, the monosynaptic EPSPs produced by sensory neurons in both interneurons and motor cells progressively decrease, paralleling the habituation of gill withdrawal. In addition, repeated stimulation also leads to a decrease in the strength of synaptic transmission from the excitatory interneurons to the motor neurons; the net result is that the reflex response diminishes (Figure 66–2B,C).
What reduces the effectiveness of synaptic transmission between the sensory neurons and their postsynaptic cells during repeated stimulation? Quantal analysis revealed that the amount of glutamate released from presynaptic terminals of sensory neurons decreases. That is, fewer synaptic vesicles are released with each action potential in the sensory neuron; the sensitivity of the postsynaptic glutamate receptors does not change. Because the reduction in transmission occurs in the activated pathway itself and does not require another modulatory cell, the reduction is referred to as homosynaptic depression. This depression lasts many minutes.
An enduring change in the functional strength of synaptic connections thus constitutes the cellular mechanism mediating short-term habituation. As change of this type occurs at several sites in the gill-withdrawal reflex circuit, memory is distributed and stored throughout the circuit. Depression of synaptic transmission by sensory neurons, interneurons, or both is a common mechanism underlying habituation of escape responses of crayfish and cockroaches as well as startle reflexes in vertebrates.
How much can the effectiveness of a synapse change and how long can the change last? In Aplysia a single session of 10 stimuli leads to short-term habituation of the withdrawal reflex lasting minutes. Four sessions separated by periods ranging from several hours to 1 day produce long-term habituation, lasting as long as 3 weeks (Figure 66–3).
Figure 66–3 Long-term habituation of the gill-withdrawal reflex in Aplysia. (Adapted, with permission, from Castellucci, Carew, and Kandel 1978.)
A. Comparison of the action potentials and synaptic potentials in sensory and motor neurons, respectively, in an untrained animal (control) and one that has been subjected to long-term habituation. In the habituated animal 1 week after training no synaptic potential occurs in the motor neuron in response to the sensory neuron action potential.
B. The mean percentage of sensory neurons making physiologically detectable connections with motor neurons in habituated animals is decreased at three points in time after long-term habituation training.
Anatomical studies indicate that long-term habituation is caused by a decrease in the number of synaptic contacts between sensory and motor neurons. In naïve animals 90% of the sensory neurons make physiologically detectable connections with identified motor neurons. In contrast, in animals trained for long-term habituation the incidence of connections is reduced to 30%; the reduction in number of synapses persists for a week and does not fully recover even 3 weeks later. As we shall see later, long-term sensitization of synaptic transmission is associated with an increase in the number of synapses between sensory and motor neurons.
Not all synapses are equally modifiable. In Aplysia the strength of some synapses rarely changes, even with repeated activation. In synapses specifically involved in learning (such as the connections between sensory and motor neurons in the withdrawal reflex circuit) a relatively small amount of training can produce large and enduring changes in synaptic strength.
Sensitization Involves Presynaptic Facilitation of Synaptic Transmission
When an animal repeatedly encounters a harmless stimulus, its responsiveness to the stimulus habituates. In contrast, with a harmful stimulus the animal typically learns fear; it responds vigorously not only to the harmful stimulus but also to other concurrent stimuli, even harmless ones. As a result, defensive reflexes for withdrawal and escape become heightened. This enhancement of reflex responses is called sensitization.
Like habituation, sensitization can be transient or long lasting. A single shock to the tail of an Aplysia produces short-term sensitization of the gill-withdrawal reflex that lasts minutes; five or more shocks to the tail produce sensitization lasting days to weeks. Tail shock is also sufficient to overcome the effects of habituation and enhance a habituated gill-withdrawal reflex, a process termed dishabituation.
Sensitization and dishabituation result from an enhancement in synaptic transmission at several connections in the neural circuit of the gill-withdrawal reflex, including the connections made by sensory neurons with motor neurons and interneurons—the same synapses depressed by habituation (Figure 66–4A). Typically, modifiable synapses can be regulated bidirectionally, participate in more than one type of learning, and store more than one type of memory. The bidirectional synaptic changes that underlie habituation and sensitization are the result of different cellular mechanisms. In Aplysia the same synapses that are weakened by habituation through a homosynaptic process can be strengthened by sensitization through a heterosynaptic process that depends on modulatory interneurons activated by the harmful stimulus to the tail.
Figure 66–4 (Opposite) Short-term sensitization of the gill-withdrawal reflex in Aplysia.
A. Sensitization of the gill-withdrawal reflex is produced by applying a noxious stimulus to another part of the body, such as the tail. A shock to the tail activates tail sensory neurons that excite facilitating (modulatory) interneurons, which form synapses on the cell body and terminals of the mechanoreceptor sensory neurons that innervate the siphon. Through these axo-axonic synapses the modulatory interneurons enhance transmitter release from the siphon sensory neurons onto their postsynaptic gill motor neurons (presynaptic facilitation), thus enhancing gill withdrawal. Presynaptic facilitation results, in part, from a prolongation of the sensory neuron action potential (bottom traces). (Adapted, with permission, from Pinsker et al. 1970; Klein and Kandel 1980.)
B. Presynaptic facilitation in the sensory neuron is thought to occur by means of two biochemical pathways. The diagram shows details of the synaptic complex in the dashed box in part A.
Pathway 1: A facilitating interneuron releases serotonin (5-HT), which binds to metabotropic receptors in the sensory neuron terminal. This action engages a G protein (Gs), which in turn increases the activity of adenylyl cyclase. The adenylyl cyclase converts ATP to cAMP, which binds to the regulatory subunit of PKA, thus activating its catalytic subunit. The catalytic subunit phosphorylates certain K+ channels, thereby closing the channels and decreasing the outward K+ current. This prolongs the action potential, thus increasing the influx of Ca2+ through voltage-gated Ca2+ channels and thereby augmenting transmitter release.
Pathway 2: Serotonin binds to a second class of metabotropic receptor that activates the Gq/11 class of G protein that enhances the activity of phospholipase C (PLC). The PLC activity leads to production of diacylglycerol, which activates protein kinase C (PKC). Phosphorylation of presynaptic proteins by PKC results in the mobilization of vesicles containing glutamate from a reserve pool to a releasable pool at the active zone, increasing the efficiency of transmitter release.
At least three groups of modulatory interneurons are involved in sensitization. The best studied use serotonin as a transmitter. The serotonergic interneurons form synapses on many regions of the sensory neurons, including axo-axonic synapses on the presynaptic terminals of the sensory cells. The serotonin released from the interneurons after a single tail shock binds to a type of receptor in the sensory neurons that is coupled to a stimulatory G protein that increases the activity of adenylyl cyclase. This action produces the second messenger cyclic adenosine monophosphate (cAMP), which in turn activates the cAMP-dependent protein kinase (PKA) (see Chapter 11). Serotonin also activates a second type of G protein-coupled receptor that leads to the hydrolysis of phospholipids and the activation of protein kinase C (PKC).
The protein phosphorylation mediated by PKA and PKC enhances the release of transmitter from sensory neurons through at least two mechanisms (Figure 66–4B). In one action PKA phosphorylates a K+ channel, causing it to close. This broadens the action potential and thus enhances Ca2+ influx through voltage-gated Ca2+ channels, which in turn enhances transmitter release. In a second action protein phosphorylation through PKC enhances the functioning of the release machinery directly. Presynaptic facilitation in response to release of serotonin by a tail shock lasts for a period of many minutes. Repeated noxious stimuli can strengthen synaptic activity for days.
Classical Conditioning of Fear Involves Coordinated Pre- and Postsynaptic Facilitation of Synaptic Transmission
Classical conditioning is a more complex form of learning. Rather than learning about the properties of one stimulus, as in habituation and sensitization, the animal learns to associate one type of stimulus with another. As described in Chapter 65, an initial weak conditioned stimulus (such as the ringing of a bell) becomes highly effective in producing a response when paired with a strong unconditioned stimulus (such as presentation of food). In reflexes that can be enhanced by both classical conditioning and sensitization, like the defensive withdrawal reflexes of Aplysia, classical conditioning results in greater and longer-lasting enhancement.
For classical conditioning of the Aplysia gill-withdrawal reflex, a weak touch to the siphon serves as the conditioned stimulus while a strong shock to the tail serves as the unconditioned stimulus. When the gill withdrawal reflex is classically conditioned, gill withdrawal in response to siphon stimulation alone is greatly enhanced. This enhancement is even more dramatic than the enhancement produced in an unpaired pathway by tail shock alone (sensitization). In classical conditioning the timing of the conditioned and unconditioned stimuli is critical. To be effective, the conditioned stimulus (siphon touch) must precede (and predict) the unconditioned stimulus (tail shock), often within an interval of about 0.5 seconds (Figure 66–5).
Figure 66–5 Classical conditioning of the gill-withdrawal reflex in Aplysia. (Adapted, with permission, from Hawkins et al. 1983.)
A. The siphon is stimulated by a light touch and the tail is shocked, but the two stimuli are not paired in time. The tail shock excites facilitatory interneurons that form synapses on the presynaptic terminals of sensory neurons innervating the mantle shelf and siphon. This is the mechanism of sensitization. 1. The pattern of unpaired stimulation during training. 2. Under these conditions the size of the motor neuron EPSP is only weakly facilitated by the tail shock. In this example the EPSP actually decreases slightly despite the tail shock because repeated unpaired stimulation of the siphon leads to synaptic depression.
B. The tail shock is paired with stimulation of the siphon. 1. The siphon is touched (conditioned stimulus or CS) immediately prior to shocking the tail (unconditioned stimulus or US). As a result, the siphon sensory neurons are primed to be more responsive to input from the facilitatory interneurons in the unconditioned pathway. This is the mechanism of classical conditioning; it both amplifies the response of the conditioned pathway and restricts the amplification to that pathway. 2. Recordings of EPSPs in an identified motor neuron produced by the siphon sensory neurons before training and one hour after training. After training the EPSP in the motor neuron produced by paired sensory input is considerably greater than either the EPSP before training or the EPSP following unpaired tail shock (shown in part A2). This produces a more vigorous gill withdrawal.
The convergence in individual sensory neurons of the signals initiated by the conditioned and unconditioned stimuli is critical. A strong shock to the tail excites serotonergic interneurons that form synapses on presynaptic terminals of the siphon sensory neurons, resulting in presynaptic facilitation associated with sensitization (Figure 66–5A). However, when the tail shock (unconditioned stimulus) immediately follows a slight tap on the siphon (conditioned stimulus), the serotonin from the interneurons produces even greater presynaptic facilitation, a process termed activity-dependent facilitation (Figure 66–5B).
How does this work? During conditioning the modulatory interneurons activated by tail shock release serotonin shortly after siphon touch has triggered an action potential in the sensory neurons. The action potential triggers an influx of Ca2+ into the sensory neuron’s presynaptic terminals, the Ca2+ binds to calmodulin, and the complex in turn binds to adenylyl cyclase. This primes the adenylyl cyclase so that it responds more vigorously to the serotonin released following the unconditioned stimulus at the tail. As a result, the production of cAMP is enhanced, which increases the amount of presynaptic facilitation. If the order of stimuli is reversed so that serotonin release precedes Ca2+ influx in the presynaptic terminals, there is no potentiation and no classical conditioning.
Thus the cellular mechanism of classical conditioning in the monosynaptic pathway of the withdrawal reflex is largely an elaboration of the mechanism of sensitization, with the added feature that the adenylyl cyclase serves as a coincidence detector in the presynaptic sensory neuron, recognizing the temporal order of the physiological representations of both the unconditioned stimulus (tail shock) and conditioned stimulus (siphon touch).
In addition to the presynaptic component of activity-dependent facilitation, a postsynaptic component is triggered by Ca+ influx into the motor neuron when it is highly excited by the siphon sensory neurons. The properties of this postsynaptic mechanism are similar to those of long-term potentiation of synaptic transmission in the mammalian brain (discussed later in this chapter and in Chapter 67).
Long-Term Storage of Implicit Memory Involves Changes in Chromatin Structure and Gene Expression Mediated by the cAMP-PKA-CREB Pathway
Cyclic AMP Signaling Has a Role in Long-Term Sensitization
In all forms of learning practice makes perfect. Repeated experience converts short-term memory into a long-term form. In Aplysia the form of long-term memory that has been most intensively studied is long-term sensitization. Like the short-term form, long-term sensitization of the gill-withdrawal reflex involves changes in the strength of connections at several synapses, including those between sensory and motor neurons. However, it also involves the growth of new synaptic connections.
Five spaced training sessions (or repeated applications of serotonin) over approximately 1 hour produce long-term sensitization and long-term synaptic facilitation lasting 1 or more days; continued spaced training over several days produces sensitization that persists for 1 or more weeks. Long-term sensitization, like the short-term form, requires protein phosphorylation that is dependent on increased levels of cAMP (Figure 66–6).
Figure 66–6 Long-term sensitization involves synaptic facilitation and the growth of new synaptic connections.
A. Long-term sensitization of the gill-withdrawal reflex of Aplysia following repeated tail shocks involves long-lasting facilitation of transmitter release at the synapses between sensory and motor neurons.
B. Long-term sensitization of the gill-withdrawal reflex leads to persistent activity of PKA, resulting in the growth of new synaptic connections. Repeated tail shock leads to more pronounced elevation of cAMP, producing long-term facilitation (lasting 1 or more days) that outlasts the increase in cAMP and recruits the synthesis of new proteins. This inductive mechanism is initiated by translocation of PKA to the nucleus (pathway 1), where PKA phosphorylates the transcriptional activator CREB-1 (cAMP response element binding protein 1) (pathway 2). CREB-1 binds cAMP regulatory elements (CRE) located in the upstream region of several cAMP-inducible genes, activating gene transcription (pathway 3). PKA also activates the mitogen-activated protein kinase (MAPK), which phosphorylates the transcriptional repressor CREB-2 (cAMP response element binding protein 2), thus removing its repressive action. One gene activated by CREB-1 encodes a ubiquitin hydrolase, a component of a specific ubiquitin proteasome that leads to the proteolytic cleavage of the regulatory subunit of PKA, resulting in persistent activity of PKA, even after cAMP has returned to its resting level (pathway 4). CREB-1 also activates the expression of the transcription factor C/EBP, which leads to expression of a set of unidentified proteins important for the growth of new synaptic connections (pathway 5).
The conversion of short-term memory into long-term memory, called consolidation, requires synthesis of messenger RNAs and proteins in the neurons in the circuit. Thus specific gene expression is required for long-term memory. The transition from short-term to long-term memory depends on the prolonged rise in cAMP that follows repeated applications of serotonin. This leads to prolonged activation of PKA, allowing the catalytic subunit of the kinase to translocate into the nucleus of the sensory neurons. It also leads indirectly to activation of a second protein kinase, the mitogen-activated protein kinase (MAPK), a kinase commonly associated with cellular growth (see Chapter 11). Within the nucleus the catalytic subunit of PKA phosphorylates and thereby activates the transcription factor CREB-1 (cAMP response element binding protein 1), which binds a promoter element called CRE (cAMP recognition element) (Figures 66–6 and 66–7).
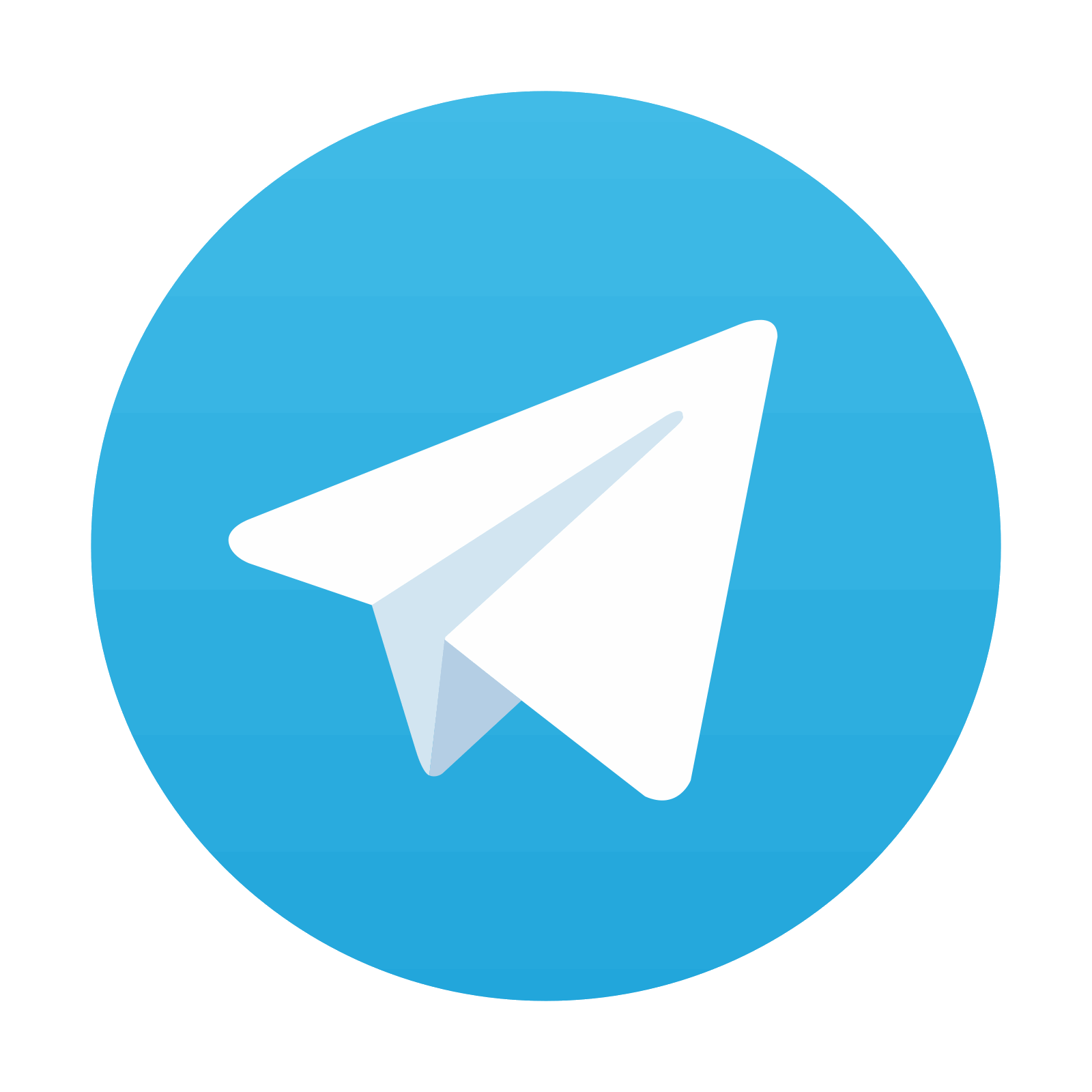
Stay updated, free articles. Join our Telegram channel
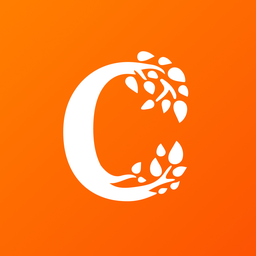
Full access? Get Clinical Tree
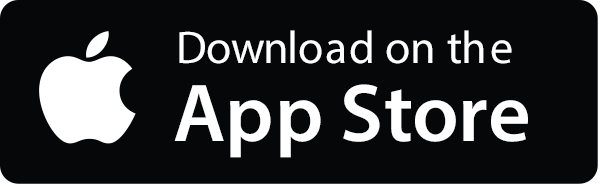
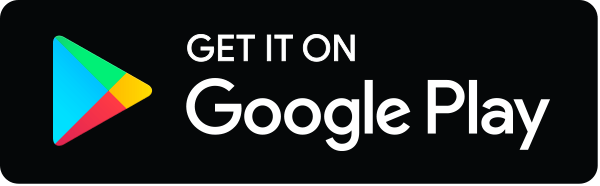