Patterning the Nervous System
The Neural Tube Becomes Regionalized Early in Embryogenesis
Secreted Signals Promote Neural Cell Fate
Development of the Neural Plate Is Induced by Signals from the Organizer Region
Neural Induction Is Mediated by Peptide Growth Factors and Their Inhibitors
Signals from the Mesoderm and Endoderm Define the Rostrocaudal Pattern of the Neural Plate
The Dorsal Neural Tube Is Patterned by Bone Morphogenetic Proteins
Dorsoventral Patterning Mechanisms Are Conserved Along the Rostrocaudal Extent of the Neural Tube
Local Signals Determine Functional Subclasses of Neurons
Rostrocaudal Position Is a Major Determinant of Motor Neuron Subtype
Local Signals and Transcriptional Circuits Further Diversify Motor Neuron Subtypes
The Developing Forebrain Is Patterned by Intrinsic and Extrinsic Influences
Inductive Signals and Transcription Factor Gradients Establish Regional Differentiation
A VAST ARRAY OF NEURONS AND GLIAL CELLS is produced during development of the vertebrate nervous system. Different neurons develop in discrete anatomical positions, acquire varied morphological forms, and establish connections with specific populations of target cells. The diversity of neurons is far greater than that of cells in any other organ of the body. The retina, for example, has dozens of classes of amacrine interneurons, and the spinal cord more than a hundred motor neuron classes. Nevertheless, the true number of neuronal classes in the mammalian central nervous system remains unclear—perhaps more than a thousand.
The diversity of neuronal cell types underlies the impressive computational properties of the mammalian nervous system. Yet, as we describe in this chapter and those that follow, the developmental principles that drive the differentiation of the nervous system are begged and borrowed from those used to direct the development in other tissues. In one sense the development of the nervous system merely represents an elaborate example of the basic challenge that pervades all of developmental biology: How to convert a single cell, the fertilized egg, into the highly differentiated cell types that characterize the mature organism.
Indeed, the convergence of developmental biology and neural science has led us to appreciate that superficial differences in the structure of the nervous systems of diverse species belie the expression of commonly shared principles and mechanisms of neural development that have been conserved throughout evolution. Much of what we know about the cellular and molecular bases of neural development in vertebrates comes from genetic studies of so-called simple organisms, most notably the fruit fly Drosophila melanogaster and the worm Caenorhabditis elegans.
Nevertheless, because the eventual goal of studies of neural development is surely to explain how the assembly of the nervous system directs and constrains human behavior, in this chapter and those that follow our description of the rules and principles of nervous system development focus primarily on vertebrate organisms.
The Neural Tube Becomes Regionalized Early in Embryogenesis
The nervous system begins to develop at a relatively late stage in the entire program of embryonic development. Well before it forms, however, the primitive embryo has generated three main cell, or germ, layers—the endoderm, mesoderm, and ectoderm.
The endoderm is the innermost germ layer that later gives rise to the gut tube, as well as to the lungs, pancreas, and liver. The mesoderm is the middle germ layer that gives rise to muscle, connective tissues, and much of the vascular system. The ectoderm is the outermost layer that gives rise to the skin as well as to the columnar epithelium of the neural plate, the precursor of the central and peripheral nervous systems.
Soon after the neural plate forms it begins to fold into a tubular structure, the neural tube, through a process called neurulation (Figure 52-1). The caudal region of the neural tube gives rise to the spinal cord, whereas the rostral region becomes the brain.
Figure 52-1 The neural plate folds to form the neural tube. (Electron micrographs of chick neural tube reproduced, with permission, from G. Schoenwolf.)
A. Early in embryogenesis three germ cell layers—the ectoderm, mesoderm, and endoderm—lie close together. The ectoderm gives rise to the neural plate, the precursor of the central and peripheral nervous systems.
B. The neural plate buckles at its midline to form the neural groove.
C. Closure of the dorsal neural folds forms the neural tube.
D. The neural tube lies over the noto-chord and is flanked by somites, an ovoid group of mesodermal cells that give rise to muscle and cartilage.
During these early stages of neural development cells divide rapidly, although cell proliferation is not uniform. Individual regions of the neural epithelium expand at different rates and begin to form the various specialized regions of the mature central nervous system. Differences in the rate of proliferation of cells in rostral regions of the neural tube result in the formation of three brain vesicles: the forebrain (or pros-encephalic) vesicle, the midbrain (or mesencephalic) vesicle, and the hindbrain (or rhombencephalic) vesicle (Figure 52-2A).
Figure 52-2 Sequential stages of neural tube development.
A. At early stages of neural tube development there are three brain vesicles.
B. Shortly after, two additional vesicles form, one in the forebrain (giving rise to regions 1a and 1b) and the other in the hindbrain (giving rise to regions 3a and 3b).
C. Top-down view of the neural tube of a chick embryo at the five-vesicle stage. (Reproduced, with permission, from G. Schoenwolf.)
D. The neural tube bends at the cephalic, pontine, and cervical flexures.
At this early three-vesicle stage the neural tube flexes twice: once at the cervical flexure, at the junction of the spinal cord and hindbrain, and once at the cephalic flexure, at the junction of the hindbrain and midbrain. A third flexure, the pontine flexure, forms later, and later still the cervical flexure straightens out and becomes indistinct (Figure 52D). The cephalic flexure remains prominent throughout development, and its persistence is the reason why the orientation of the longitudinal axis of the forebrain deviates from that of the brain stem and spinal cord.
As the neural tube develops, two of the primary embryonic vesicles divide further, thus forming the five-vesicle stage (Figure 52–2B, C). The forebrain vesicle divides to form the telencephalon and diencephalon, and the hindbrain vesicle divides to form the metencephalon and myelencephalon. Together with the spinal cord these subdivisions make up the major functional regions of the mature central nervous system (see Chapter 15). These functional domains are the products of progressive patterning and subdivision of the neural tube, developmental events that are regulated by a variety of secreted signals.
Secreted Signals Promote Neural Cell Fate
As with other organs, the emergence of the neural plate is the culmination of a complex molecular program that involves the tightly orchestrated expression of specific genes within ectodermal cells. The entire nervous system derives from a restricted region of the ectoderm.
Early in development ectodermal cells are faced with the choice of whether to become neural or epidermal cells. This decision is arguably the most fundamental step of neural development, and one that has been the subject of intense study for nearly 100 years. Much of this interest has focused on a search for signals that control the fate of ectodermal cells.
We now know that two major classes of proteins work together to promote the differentiation of an ectodermal cell into a neural cell. The first are inductive factors, signaling molecules that are secreted by nearby cells. Some of these factors are freely diffusible and exert their actions at a distance, but others are tethered to the cell surface and act locally. The second are surface receptors that enable cells to respond to inductive factors. Activation of these receptors triggers the expression of genes that encode intracellular proteins—transcription factors, enzymes, and cytoskeletal proteins—which push ectodermal cells along the pathway to becoming neural cells.
The ability of a cell to respond to inductive signals, termed its competence, depends on the exact repertory of receptors, transduction molecules, and transcription factors expressed by the recipient cell. Thus a cell’s fate is determined not only by the signals to which it is exposed—a consequence of when and where it finds itself in the embryo—but also by the profile of genes it expresses as a consequence of its prior developmental history. We will see in subsequent chapters that the interaction of localized inductive signals and intrinsic cell responses is evident at virtually every step throughout neural development.
Development of the Neural Plate Is Induced by Signals from the Organizer Region
The discovery that specific signals are responsible for triggering the formation of the neural plate was the first major advance in understanding the mechanisms that pattern the nervous system. In 1924 Hans Spemann and Hilde Mangold made the remarkable observation that the differentiation of the neural plate from uncommitted ectoderm depends on signals secreted by a specialized group of cells they called the organizer region.
Working with amphibian embryos they showed that organizer activity is restricted to a region of the embryo called the dorsal lip of the blastopore, which is destined to form the dorsal mesoderm. Spemann and Mangold demonstrated the crucial role of the organizer in forming the nervous system by transplanting small pieces of dorsal blastopore lip tissue underneath the ventral ectoderm of a host embryo, a region that normally gives rise to ventral epidermal tissue (Figure 52-3). By grafting organizer cells from a pigmented embryo into an unpigmented host, they were able to distinguish the position and fate of donor and host cells.
Figure 52-3 Signals from the organizer region induce a second neural tube. (Micrographs reproduced, with permission, from Eduardo de Robertis.)
Left: In the normal frog embryo cells from the organizer region (the dorsal blastopore lip) populate the notochord, floor plate, and somites. Right: Spemann and Mangold grafted the dorsal blastopore lip from an early gastrula stage embryo into a region of a host embryo that normally gives rise to the ventral epidermis. Signals from grafted cells induce a second embryonic axis, which includes a virtually complete neural tube. The donor tissue was from a pigmented embryo, whereas the host tissue was unpigmented, permitting the fate of grafted cells to be monitored by their characteristic pigmentation. Grafted cells themselves contribute only to the notochord, floor plate, and somites of the host embryo. As the embryo matures the secondary neural tube develops into a complete nervous system. In the Xenopus embryo shown in the micrograph, the second neural axis was induced by injection of an antagonist of bone morphogenetic protein (BMP), in effect substituting for the organizer signal (Figure 52-4). The primary neural axis is also apparent. (V, ventral; D, dorsal.)
Spemann and Mangold found that transplanted organizer cells followed their normal developmental program, generating midline mesoderm tissue such as the somites and notochord. But the transplanted cells also caused a striking change in the fate of the neighboring ventral ectodermal cells of the host embryo. Host ectodermal cells were induced to form a virtually complete copy of the nervous system (Figure 52-3). Spemann and Mangold went on to show that organizer cells were the only tissue that possessed this inductive effect.
These pioneering studies revealed that the nervous system is induced by signals from a highly restricted organizing center. As we will discuss, many aspects of neural tube patterning are now known to depend on signals secreted by other local organizing centers through actions similar in principle to that of the classical organizer region.
Neural Induction Is Mediated by Peptide Growth Factors and Their Inhibitors
For decades after Spemann and Mangold’s pioneering studies, identification of the neural inducer constituted a Holy Grail of developmental biology. The search was marked by little success until the 1980s, when the advent of molecular biology and the availability of better markers of early neural tissue led to a breakthrough in our understanding of neural induction and its chemical mediators.
The first advance came from a simple finding: when the early ectoderm is dissociated into single isolated cells, effectively preventing cell-to-cell signaling, the cells readily acquire neural properties in the absence of added factors. The surprising implication of this finding was that the “default” fate of ectodermal cells is neural differentiation and that this fate is prevented by signals from neighboring ectodermal cells. In other words, the long sought-after “inducer” is actually a “de-repressor” of neural fate.
These ideas immediately raised two further questions. What ectodermal signal represses neural differentiation and what does organizer tissue provide to overcome the effects of the repressor? Studies of neural induction in frogs and chicks have now provided partial answers to these questions.
In the absence of signals from the organizer, ectodermal cells synthesize and secrete bone morphogenetic proteins (BMP), members of a large family of transforming growth factor β (TGFβ) related proteins. The BMPs, acting through serine/threonine kinase class receptors on ectodermal cells, suppress the potential for neural differentiation and promote epidermal differentiation. Key evidence for the role of BMPs as neural repressors came from experiments in which a truncated version of a BMP receptor, which blocks BMP signaling, was found to trigger the differentiation of neural tissue in the Xenopus frog embryo. Conversely, exposure of ectodermal cells to BMP signaling promoted differentiation as epidermal cells (Figure 52-4).
Figure 52-4 Inhibition of bone morphogenetic protein (BMP) signaling initiates neural induction.
A. In Xenopus frog embryos signals from the organizer region (red line) spread through the ectoderm to induce neural tissue. Ectodermal tissue that is beyond the range of organizer signals gives rise to epidermis.
B. BMP inhibitors secreted from the organizer region (including noggin, follistatin, and chordin) bind to BMPs and block the ability of ectodermal cells to acquire an epidermal fate, thus promoting neural character.
C. Ectodermal cells acquire neural or epidermal character depending on the presence or absence of BMP signaling. When ectodermal cell aggregates are exposed to BMP signaling they differentiate into epidermal tissue. When BMP signaling is blocked, either by dissociating ectodermal tissue into single cells or by addition of BMP inhibitors to ectodermal cell aggregates, the cells differentiate into neural tissue.
The identification of BMPs as suppressors of neuronal differentiation in turn raised the possibility that the ability of organizer tissue to induce neural differentiation in ectodermal cells might be mediated by factors that antagonize BMP signaling. Direct support for this idea came from the finding that cells of the organizer region express many secreted proteins that act as BMP antagonists. These proteins include noggin, chordin, follistatin, and even some variant BMP proteins. Each of these proteins has the ability to induce ectodermal cells to differentiate into neural tissue. Thus there is no single neural inducer. In fact, multiple classes of proteins are required for induction, as shown by the later finding that the exposure of ectodermal cells to fibroblast growth factors (FGFs) is also a necessary step in neural differentiation.
Together these studies have provided a molecular explanation of the cellular phenomena first described by Spemann and Mangold. Nevertheless, many details of the pathway of neural induction remain to be clarified. We know that transcription factors of the SoxB family, expressed in prospective neural plate cells, function as intermediaries in the acquisition of neural character. But other components of the pathway remain to be uncovered.
Rostrocaudal Patterning of the Neural Tube Involves Signaling Gradients and Secondary Organizing Centers
As soon as cells of the neural plate have been induced they begin to acquire regional characteristics that mark the first steps in the differentiation of the forebrain, midbrain, hindbrain, and spinal cord. Cells in each of these four major regions acquire diverse neuronal fates and identities.
The subdivision of the neural plate into its major functional domains is directed by a series of secreted inductive factors and follows the same basic principles of neural induction. These inductive factors are initially secreted from mesodermal and endodermal cells that flank the neural plate. Later, after neural tube closure, they are also secreted from secondary organizing centers embedded within the neural tube. Some of these factors generate a broad rostrocaudal signaling gradient that can span the entire neural plate whereas others act more locally.
Neural plate cells in different regions of the neural tube respond to these inductive signals by expressing distinct transcription factors that gradually constrain the developmental potential of cells in each local domain. In this way neurons at different rostrocaudal levels acquire distinct identities and functions and the neural tube becomes subdivided along its rostrocaudal axis into functionally specific domains.
Signals from the Mesoderm and Endoderm Define the Rostrocaudal Pattern of the Neural Plate
The rostrocaudal patterning of the neural plate is initiated by factors secreted by mesodermal and endodermal tissues that flank the neural plate. One important class of factors is the Wnt proteins (an acronym based on their founding family members, the Drosophila Wingless protein and the mammalian Int1 proto-oncogene protein).
The net level of Wnt signaling activity is low at rostral levels of the neural plate and increases progressively in the caudal direction. This activity gradient arises because the mesoderm that flanks caudal regions of the neural plate expresses high levels of Wnt, whereas the endoderm that underlies the rostral region of the neural plate is a source of secreted proteins that inhibit Wnt signaling, much as BMP inhibitors attenuate BMP signaling at an earlier stage. Cells at progressively more caudal positions along the neural plate are exposed to increasing levels of Wnt activity and thus acquire a more caudal regional character, spanning the entire range from forebrain, to midbrain, to hindbrain and finally to spinal cord (Figure 52-5).
Figure 52-5 Early anteroposterior patterning signals establish distinct transcription factor domains and define the position of the midbrain-hindbrain boundary region. The anteroposterior pattern of the neural plate is established by exposure of neural cells to a gradient of Wnt signals. Anterior (A) regions of the neural plate are exposed to Wnt inhibitors secreted from the endoderm and thus perceive only low levels of Wnt activity. Progressively more posterior (P) regions of the neural plate are exposed to high levels of Wnt signaling from the paraxial mesoderm and to lower levels of Wnt inhibitors. In response to this Wnt signaling gradient and other signals, cells in anterior and posterior regions of the neural plate begin to express different transcription factors: Otx2 at anterior levels and Gbx2 at more posterior levels. The intersection of these two transcription factor domains marks the region of the midbrain-hindbrain boundary (MHB), where Engrailed transcription factors are expressed. (Adapted, with permission, from Wurst and Bally-Cuif 2001.)
After the neural tube has acquired its initial rostrocaudal character, the mesoderm and endoderm secrete additional signals that further refine this pattern. At the very rostral margin of the neural tube a specialized group of cells, called the anterior neural ridge, secretes FGF that patterns the telencephalon, as we discuss later in the chapter. At more caudal levels of the neuraxis the secretion of retinoic acid and FGF from the mesoderm establishes distinct subdomains of the hindbrain and spinal cord.
Signals from Organizing Centers within the Neural Tube Pattern the Forebrain, Midbrain, and Hindbrain
The early influence of mesodermal and endodermal tissues on rostrocaudal neural pattern is further refined by signals from two specialized cell groups in the neural tube itself. One of these cell groups is called the zona limitans intrathalamica and appears as a pair of horn-like spurs within the diencephalon (Figure 52-6). Zona limitans intrathalamica cells secrete the protein sonic hedgehog (Shh), which patterns nearby cells that give rise to the nuclei of the thalamus. (The actions of sonic hedgehog are described in detail below in the context of its prominent role in spinal cord patterning.)
Figure 52-6 Local signaling centers in the developing neural tube. (Adapted, with permission, from Kiecker and Lumsden 2005.)
A. This side view of the early neural tube shows the location of the midbrain-hindbrain boundary (MHB), the source of secreted signals that pattern cell types in the midbrain and hindbrain. The prechordal plate and notochord are two nonneural signaling centers that influence the dorsoventral patterning of the neural tube.
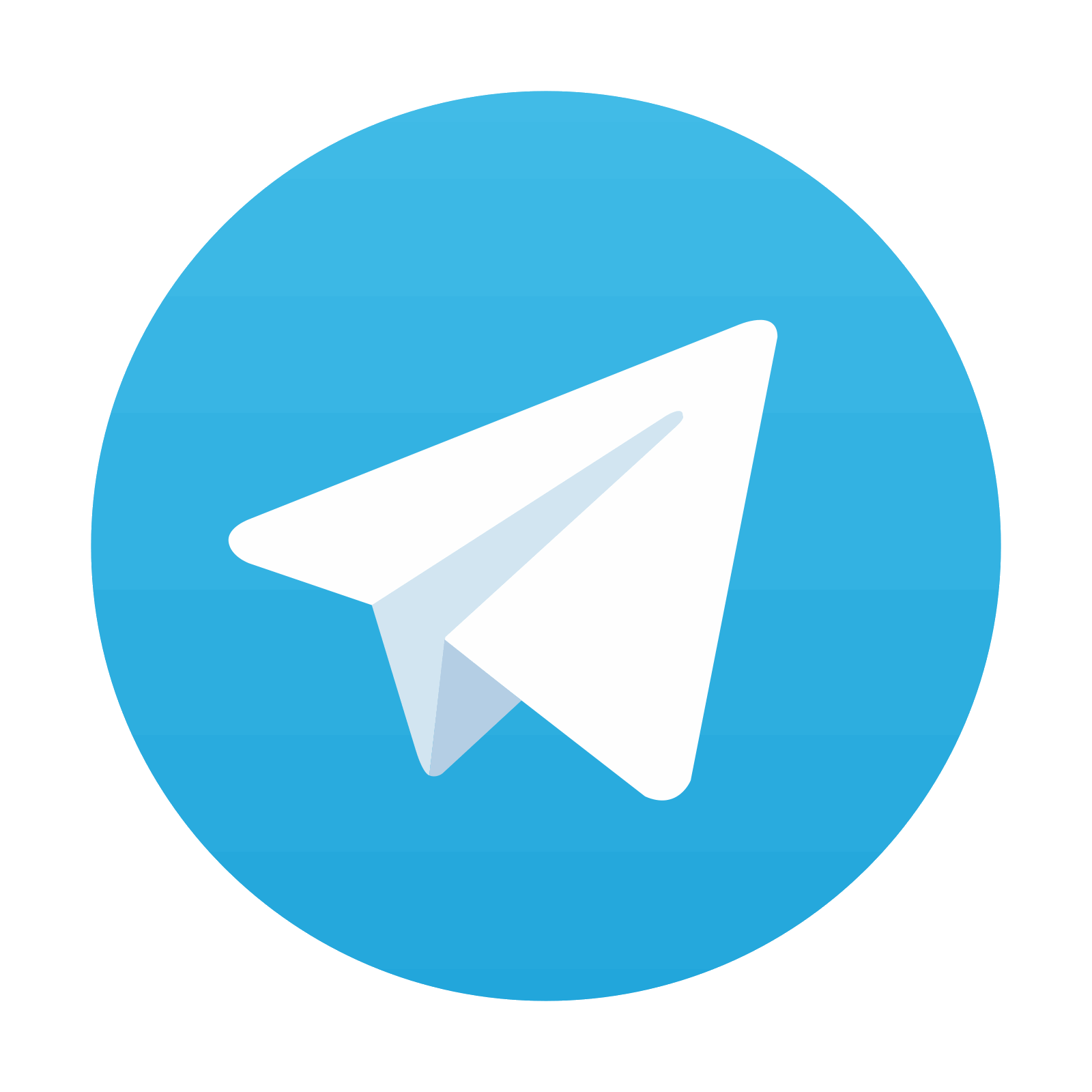
Stay updated, free articles. Join our Telegram channel
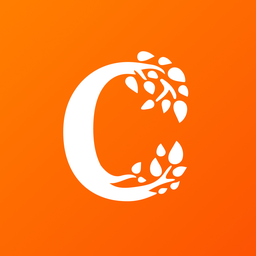
Full access? Get Clinical Tree
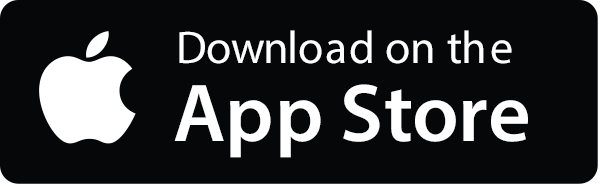
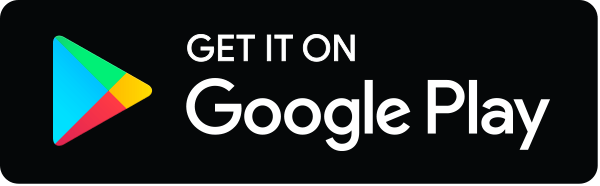