Abstract
Copper is one of the six transition metals that have important biochemical roles in humans, particularly in catalysis and electron transport [1, 2]. Because it can exist in two redox states (Cu2+/Cu+), it can participate in redox reactions involving transfer of an electron, but if it builds up it can also generate potentially toxic reactive oxygen species by Fenton chemistry. Examples of copper in redox enzymes include: complex IV of the mitochondrial respiratory chain, copper–zinc superoxide dismutase, ceruloplasmin (ferroxidase), lysyl oxidase, dopamine beta-hydroxylase, and tyrosinase.
Copper Metabolism and Transport, Deficiency, and Toxicity
Copper is one of the six transition metals that have important biochemical roles in humans, particularly in catalysis and electron transport [1, 2]. Because it can exist in two redox states (Cu2+/Cu+), it can participate in redox reactions involving transfer of an electron, but if it builds up it can also generate potentially toxic reactive oxygen species by Fenton chemistry. Examples of copper in redox enzymes include: complex IV of the mitochondrial respiratory chain, copper–zinc superoxide dismutase, ceruloplasmin (ferroxidase), lysyl oxidase, dopamine beta-hydroxylase, and tyrosinase.
Copper is present in many foods and in drinking water. The content is particularly high in organ meats (e.g. liver), shellfish, chocolate, nuts, and mushrooms. The content in food may be increased by cooking in copper-containing vessels. Copper absorption is reduced by gastric bypass surgery and this can lead to a myelopathy [3].
Copper is transported into the enterocyte via the CTR1 transporter. Export from the enterocyte is regulated by the copper-transporting ATPase, ATP7A. This protein is synthesized in the endoplasmic reticulum and resides in the trans-Golgi network, but, as intracellular copper levels rise, it translocates to the basolateral membrane where it allows copper export into the plasma. Mutations in ATP7A give rise to generalized copper deficiency, deficient activity of copper enzymes, and the symptoms of Menkes syndrome and variants [4].
On arriving at the liver, copper is taken up through CTR1 at the basolateral membrane and rising copper levels lead to translocation of ATP7B from the trans-Golgi network to the apical membrane where the copper is excreted into the bile canaliculus [4]. ATP7B is also required for the secretion of copper bound to ceruloplasmin from the liver into plasma. Mutations in ATP7B (Wilson disease) cause an accumulation of copper in the liver with reduced plasma concentrations of ceruloplasmin and ceruloplasmin-bound copper (normally the major fraction of plasma copper). As the disease progresses, levels of free copper in the plasma increase; urinary copper excretion is higher than normal. Copper deposition in the basal ganglia of the brain is responsible for the movement disorder, copper deposition in Descemet’s membrane at the corneoscleral junction in the eye give rise to Kayser–Fleischer rings, copper deposition in the kidneys can cause a tubulopathy, and high free plasma copper can cause hemolysis.
Within the cell, chaperones are important in conveying copper to copper-dependent enzymes such as copper–zinc superoxide dismutase (chaperone: CCS) and complex IV of the respiratory chain (chaperones: SCO1 and SCO2). SCO1 mutations in the mouse lead to severe cellular copper deficiency because, in addition to its role in complex IV assembly, SCO1 is required for expression of CTR1 and hence copper uptake [5].
Copper Toxicity Disorders
Wilson Disease (ATP7B Mutations)
Wilson disease is an autosomal-recessive disorder caused by bi-allelic mutations in the ATP7B gene on chromosome 13q14.3. The incidence is approximately 1 in 30,000 live births. As discussed, there is a failure of excretion of copper from the liver into the bile and of ceruloplasmin-bound copper into the plasma. The build-up of copper in the liver causes inflammation and fibrosis. Copper is deposited in the brain (particularly the basal ganglia), the eye (Kayser–Fleischer rings), and the kidney (tubulopathy).
In a series of 400 adult patients with Wilson disease, 50% presented with neurological and psychiatric symptoms, 20% with neurological and hepatic symptoms, and 20% with purely hepatic symptoms [6].
In patients with neurological presentations, movement disorders are prominent. Dysarthria (speech difficulty) and dysgraphia are particularly characteristic. Major movement disorders include dystonia, rigidity, tremor, and choreiform movements.
Dystonia is common, present in about two-thirds of patients, and can be focal, multifocal, or generalized as the disease progresses. Focal forms of dystonia include blepharospasm, cervical dystonia, or risus sardonicus. With orofacial dystonia or oropharyngeal dyskinesia, patients may develop dysphonia, dysarthria, or dysphagia. Parkinsonism and ataxia are also found in Wilson disease although they are rarely an isolated clinical feature and are typically accompanied by other neurological deficits. Chorea is more common in children and adolescents with Wilson disease.
In patients with psychiatric presentations, common features include changes in personality (irritability, anger, poor self-control), depression, and anxiety. Frank psychosis can occur.
Ninety-five percent of patients with neurological signs have Kayser–Fleischer rings. Slit lamp examination may be required to visualize the golden/greenish-brown pigmentation in the early stages. It starts as a superior crescent and progresses inferiorly to become circumferential.
T2-weighted and fluid-attenuated inversion recovery MRI images typically show changes in the striatum but abnormalities can be present in other brain regions (Figure 17.1). In one recent series the incidence of abnormalities (percentage of patients) was: putamen, 85.3%; caudate, 67.6%; brainstem and globus pallidus, 61.8% each; thalamus, 58.8%; cerebral cortex, 26.5%; subcortical white matter, 23.5%; and cerebellum, 5.9% [7]. Both hyperintense and hypointense signals can be seen. Choreoathetosis correlated with thalamic, pallidal, and putaminal lesions; mini mental state examination, with subcortical white matter changes. MRI load correlated with age, tremor, psychiatric disorder, choreoathetosis, and severity of Wilson disease [7].
Figure 17.1 Representative cranial MRI findings in patients with Wilson disease having neurological manifestation in axial section. (a) Basal ganglia T2 hyperintensity (putamen, 85.3%; caudate, 67.6%; globus pallidus, 61.8% of patients). (b) Thalamus (58.8% of patients) and corpus striatum T2 hyperintensity. (c) Midbrain T2 hyperintensity (61.8% of patients). (d) Pontine T2 hyperintensity (61.8% of patients). (e) Frontoparietal subcortical white matter (23.5% of patients) and cortical (26.5% of patients) T2 hyperintensity. (f) Cortical atrophy in T1 sequence (20.5% of patients). From: Ranjan A, Kalita J, Kumar S et al. A study of MRI changes in Wilson disease and its correlation with clinical features and outcome. Clin Neurol Neurosurg 2015;138:31–6. Reproduced with permission.
Laboratory investigations usually show abnormalities of liver function tests (even with a pure neurological presentation) although the levels of transaminases tend to be lower than other causes of chronic hepatitis (e.g. autoimmune). Clotting times may be prolonged. Plasma copper and ceruloplasmin are low; urinary excretion of copper is elevated (Table 17.1).
A liver biopsy shows chronic inflammation with fibrosis, with increased staining for copper-associated protein. The liver copper content is increased (Table 17.1).
Many different mutations in the ATP7B gene have been described (>500). Most patients are compound heterozygotes. R778L is a common mutation in Asian patients whereas H1069Q is a common mutation in Europeans. R778L causes complete loss of function of the copper ATPase; homozygotes present early with hepatic disease. H1069Q mutations are associated with some residual activity and homozygotes present with neurological disease at around 21 years of age [4].
The treatment options for Wilson disease include drugs that chelate copper and increase its excretion, and zinc. Zinc induces metallothionein synthesis in the intestinal epithelium and the metallothionein binds copper in the villus cells (preferentially over zinc). This reduces copper absorption and increases losses into the feces as the villus cells are shed into the intestinal lumen.
Treatment of neurological Wilson disease presents a major challenge. It may save the patient’s life but make their movement disorder significantly worse and in 34% of cases where neurological deterioration occurs, it is irreversible. A recent study confirmed that worsening of neurological symptoms can occur in 35% of patients treated with D-penicillamine (n = 35) and 19% of patients treated with zinc sulphate (n = 21), a difference that was not statistically significant [8, 9]. On the other hand, a study in 2006 by Brewer et al. showed that neurological deterioration on treatment occurred in 27% of patients treated with trientine (n = 23) but in only 4% of patients treated with ammonium tetrathiomolybdate (n = 25), a difference that was significant at the p < 0.05 level [10]. Bis-choline tetrathiomolybdate is currently in a Stage III clinical trial (ClinicalTrials.gov).
Hepatic Wilson disease may require treatment of acute liver failure or end-stage chronic liver disease by liver transplantation. Liver transplantation for neurological Wilson disease remains controversial; there is a wide spectrum of outcomes post liver transplant – some with neurological recovery and others with continued disability and an overall increased mortality [11].
MEDNIK Syndrome
MEDNIK syndrome is an autosomal-recessive disorder caused by bi-allelic mutations in AP1S1, which encodes a protein that is needed for translocation of ATP7A and ATP7B from the trans-Golgi network to the cell membrane and other organelles. Plasma copper and ceruloplasmin concentrations are reduced but liver copper is elevated. The clinical features are those constituted in the acronym MEDNIK: “Mental retardation, Enteropathy, Deafness, peripheral Neuropathy, Ichthyosis, and Keratoderma” plus brain atrophy and cholestatic hepatopathy. Although brain atrophy is the most common finding on MRI, three patients have been described with symmetrical T2 hyperintensity of the basal ganglia, mainly affecting the caudate and putamen. However, a specific movement disorder has not been described. The disorder responds to treatment with zinc acetate [12, 13].
Copper Deficiency Disordes
Menkes Disease, Occipital Horn Syndrome, X-Linked Distal Hereditary Motor Neuropathy (ATP7A Mutations)
The ATP7A gene is on the X-chromosome, so mutations give rise to X-linked disorders. Menkes disease is the most severe form; the milder forms are the occipital horn syndrome and X-linked distal hereditary motor neuropathy [4].
Many of the manifestations of Menkes syndrome can be understood in terms of the effect of copper deficiency on copper-dependent enzymes (see below). The reduced activity of complex IV of the respiratory chain, copper–zinc dismutase, and ceruloplasmin probably contribute to neurological disease; the reduced cross-linking of elastin and of collagen by lysyl oxidase contribute to cutis laxa, tortuosity of the arteries, and bladder and bowel diverticulae; the reduced activity of dopamine beta-hydroxylase impairs synthesis of adrenaline and noradrenaline and contributes to hypothermia and orthostatic hypotension; and the reduced activity of tyrosinase contributes to pallor of skin and hair.
Menkes disease occurs with an incidence of approximately 1 in 250,000 live births and is a progressive neurodegenerative disorder. Major neurological signs are hypotonia progressing to hypertonia and epilepsy.
Infants with Menkes syndrome may be born prematurely and some exhibit a large cephalhematoma and/or skin laxity. The hair breaks easily. Hypothermia can be a problem in the neonatal period. By 1–2 months, hypotonia is apparent as is the characteristic appearance with sagging cheeks and loose skin over the neck. Examination of the hair under the microscope reveals the characteristic pili torti. Feeding difficulties, chronic diarrhea, and failure to thrive are common problems although linear growth is usually preserved. Seizures tend to start at about 2 months. Many seizure types have been described, including infantile spasms with hypsarrhythmia on EEG. During the first year of life hypotonia is replaced by hypertonia, and a delay in achieving motor milestones becomes increasingly apparent. In one series from China, all patients were reported to have dystonia [14]. Urinary-tract infections are common and may be difficult to treat because of bladder diverticulae; chest infections are also common. Umbilical and inguinal hernias occur frequently as does pectus excavatum. In the past, most patients died before the age of 3 years of infections or vascular complications, although with good care, especially attention to feeding, better survival could be achieved.
Skeletal X-rays often show osteopenia and Wormian bones in the skull. MRI shows cerebral atrophy, ventriculomegaly, and cerebellar atrophy at first imaging in the majority of patients, and focal lesions in the basal ganglia also occur early in the course of the disease, between 2 months and 16 months of age [15]. These lesions are typically asymmetrical and involve the caudate and anterior putamen; they are hyperintense on T2-weighted images. Subdural collections are seen in under one-fifth of scans.
Serum copper is <11 μg/dL and ceruloplasmin <200 mg/L but low values like this can be seen in the first few months of life in normal (and especially premature) infants. The abnormalities of catecholamines and their metabolites provide better diagnostic markers in young infants. Examples of useful diagnostic parameters are the ratios in plasma of dopamine to noradrenaline or of dihydroxyphenylacetic acid to dihydroxyphenylglycol or the ratio of homovanillic acid (HVA) to vanillylmandelic acid (VMA) in the urine [16]. The latter has been proposed as a test that could be used for neonatal screening; early diagnosis is important for treatment to be successful.
Treatment with parenteral copper, usually in the form of copper histidine, can improve neurological outcomes. However, some patients show no significant improvement despite early treatment initiation [17].
The occipital horn syndrome is a milder form of Menkes syndrome seen in about 10% of cases. The connective-tissue abnormalities are similar to those seen in Menkes but development is much less affected. Exostoses are palpable at the sites of some muscle insertions; the occipital horn is at the site of insertion of the paraspinal muscles. Skin and joint laxity are common problems as are the bladder diverticulae. Chronic diarrhea and orthostatic hypotension are probably both consequences of dysautonomia, particularly impaired synthesis of adrenaline and noradrenaline.
The mildest phenotype caused by ATP7A mutations is X-linked distal hereditary motor neuropathy. Affected individuals present in late childhood/adult life with weakness associated with distal muscular atrophy.
All three phenotypes of ATP7A deficiency show an X-linked mode of inheritance but approximately one-third of patients have a new mutation. The mutations producing Menkes disease vary considerably from chromosomal abnormalities (principally X-autosome translocations) through intragenic deletions involving more than one exon to single base-pair changes. These mutations are predicted to lead to a non-functional protein. In contrast, the occipital horn syndrome is usually caused by splice site mutations that permit small amounts of ATP7A protein to be produced and X-linked distal hereditary motor neuropathy is caused by a small number of missense mutations with even higher residual ATP7A activity [4].
Disorders of Specific Copper-Dependent Enzymes or Chaperones
Aceruloplasminemia
Aceruloplasminemia is an autosomal-recessive disorder caused by bi-allelic mutations in the CP gene encoding the copper-dependent enzyme ceruloplasmin [18]. Ceruloplasmin is undetectable in plasma. Patients have an accumulation of iron in the liver, islets of Langerhans, and brain. They present in adulthood with neurological symptoms (chorea, ataxia, dystonia, parkinsonism, and psychiatric disorders), retinal degeneration, and diabetes mellitus. Ceruloplasmin is a ferroxidase enzyme that converts ferrous iron (Fe2+) into ferric iron (Fe3+). It is believed to also be important in the conversion of Mn2+ to Mn3+ (see below). Patients have low serum iron (mostly transferrin-bound Fe3+), high serum ferritin (produced from excess cellular Fe2+), and low serum copper. Hepatic iron is increased.
Aceruloplasminemia is normally classified as one of the neurodegeneration with brain iron accumulation (NBIA) group and is discussed in Chapter 16.
Treatment with iron chelation and fresh frozen plasma may be useful to reduce the iron load in the central nervous system and to improve the neurological symptoms [19].
Deficiency of the Copper Chaperone for Superoxide Dismutase
The copper chaperone for superoxide dismutase (CCS) acts as a copper chaperone, delivering the metal to the copper–zinc superoxide dismutase (SOD1) enzyme. One patient with Huppke–Brendel syndrome and a homozygous truncating mutation in the SLC33A1 gene, who was reported as having congenital cataracts, hearing loss, and neurodegeneration (see the next section), also had a variant of unknown significance in CCS [20]. The patient with the CCS variant had additional symptoms not present in the other patients with SLC33A1 mutations, including neonatal hypotonia, hypoglycemia, and a pericardial effusion. At age 18 months, he had rapid developmental regression and epilepsy with persistent bilateral thalamic lesions on brain MRI. The activity of SOD1 was reduced in the fibroblasts.
Deficiency of Copper–Zinc Superoxide Dismutase
Mutations in SOD1 encoding the copper–zinc superoxide dismutase enzyme cause amyotrophic lateral sclerosis (motor neuron disease) [21]. This suggests that a reduced activity of this enzyme caused by copper deficiency may contribute to the motor neuropathy of X-linked distal motor neuropathy.
Deficiency of the Cytochrome C Oxidase Assembly Protein SCO1
SCO1 is a copper chaperone involved in the assembly of complex IV of the mitochondrial respiratory chain and also plays a role in copper homeostasis. Bi-allelic mutations cause neonatal-onset hepatic failure and encephalopathy (with or without hypertrophic cardiomyopathy), with profound lactic acidosis and reduced activity of complex IV in the liver and muscle [22]. Affected infants are profoundly hypotonic. A patient who survived to 4 months initially had very poor truncal tone with increased peripheral tone but progressed to dystonic posturing [23].
Deficiency of the Cytochrome C Oxidase Assembly Protein SCO2
SCO2 is a paralogue of SCO1 and it also participates in the assembly of complex IV of the mitochondrial respiratory chain and in copper homeostasis. Bi-allelic mutations in SCO2 cause neonatal encephalo-cardiomyopathy [24, 25]. Profound hypotonia and dystonia may be apparent. Recently, it has been shown that bi-allelic mutations in SCO2 can also cause an axonal polyneuropathy with predominantly motor involvement [26]. Affected individuals have evidence of cellular copper deficiency.
Dopamine Beta-Hydroxylase
Bi-allelic mutations in DBH cause the isolated failure of autonomic noradrenergic neurotransmission because a defect in the beta-hydroxylation of dopamine in peripheral nerves leads to a failure of synthesis of adrenaline and noradrenaline [27]. The main symptom is orthostatic hypotension. Hypothermia and hypoglycemia can occur in infancy. In two patients with lifelong orthostatic hypotension due to DBH deficiency, the oral administration of DL-dihydroxyphenylserine led to remarkable improvement [28].
Lysyl Oxidase
Heterozygous mutations in the LOL gene encoding lysyl oxidase cause autosomal-dominant familial thoracic aortic aneurysms [29].
Tyrosinase
Bi-allelic mutations in the TYR gene encoding tyrosinase cause autosomal-recessive oculocutaneous albinism [30]. Tyrosinase catalyzes the first two steps, and at least one subsequent step, in the conversion of tyrosine to melanin.
Disorders with Secondary Effects on Copper Levels
SLC33A1 Mutations/AT1 Deficiency/Huppke–Brendel Syndrome, and Autosomal-Dominant Hereditary Spastic Paraplegia 42
Mutations in SLC33A1 lead to a deficiency of the acetyl-coenzyme A (CoA) transporter AT1 that is required for entry of acetyl-CoA into the lumen of the Golgi apparatus where it participates in many acetylation reactions involving proteins and their glycans. The impaired synthesis and/or secretion of ceruloplasmin leads to low serum copper and ceruloplasmin. There is no evidence of copper deficiency or copper toxicity.
Homozygous mutations in SLC33A1 have been reported in children with autosomal-recessive congenital cataracts, hearing loss, and neurodegeneration (Huppke–Brendel syndrome) [31]. Heterozygous mutations in SLC33A1 have been described in autosomal-dominant hereditary spastic paraplegia type 42 [32].
Manganese Metabolism and Transport, Toxicity and Deficiency
Manganese is another of the six transition metals essential for human metabolism. It participates in group transfer reactions such as phosphorylation and glycosylation. Deficiency can lead to defective glycosylation of serum proteins such as transferrin. It can exist in a number of oxidation states; Mn2+ and Mn3+ are important in the body and their interconversion can facilitate redox reactions including manganese superoxide dismutase, the important mitochondrial scavenger of reactive oxygen species. Other enzymes for which manganese is a cofactor are involved in amino acid metabolism (e.g. arginase), lipid and carbohydrate metabolism, immune function, bone and connective-tissue growth, and blood clotting [33, 34].
Manganese is present in water supplies and in many foods. Foods particularly rich in manganese include cloves, saffron, nuts, mussels, dark chocolate, sesame, and sunflower seeds [35].
Uptake of manganese (Mn2+) into cells can be facilitated by a number of transporters including SLC39A8 and SLC39A14, divalent metal transporter 1 (DMT1; SLC11A2), dopamine transporter (DAT), and citrate transporters [2, 36]. Iron competes with manganese for uptake by DMT1 and also at several other stages of manganese metabolism (e.g. binding to transferrin). This explains why increasing oral iron uptake can be used in the treatment of hypermanganesemia [37, 38]. Uptake by SLC39A8 probably also contributes to the uptake of manganese from the gut and the uptake of manganese into the cells that need it; hence, SLC39A8 deficiency leads to low plasma manganese levels and signs of cellular manganese deficiency, e.g. impaired glycosylation of transferrin [39–41]. On the other hand, the uptake of manganese into the liver, facilitated by SLC39A14, appears to be important in facilitating the biliary excretion of manganese; SLC39A14 deficiency is a cause of hypermanganesemia and deposition of manganese in the brain [42].
After uptake of Mn2+ from the gut, it is oxidized in the blood by ceruloplasmin to Mn3+, which is then bound to transferrin, the major manganese-binding protein. Uptake of transferrin-bound Mn3+ occurs when it binds to the transferrin receptor and is internalized in an endocytotic vesicle (receptor-mediated endocytosis). In the endosome, Mn3+ is reduced to Mn2+ and uptake into the cytoplasm probably occurs mainly via DMT1 [36]. Stable tissue concentrations of manganese are maintained by tight homeostatic control of intestinal absorption and biliary excretion. When blood levels of manganese are elevated manganese may be deposited in the brain, particularly in the basal ganglia. Affected areas of the brain can be visualized as they produce a hyperintense signal on T1-weighted MRI [42]. Brain manganese accumulation leads to a condition known as manganism, an extrapyramidal movement disorder characterized by dystonia, bradykinesia, and rigidity, accompanied by psychiatric and cognitive defects [42].
Manganese neurotoxicity has been attributed to impaired dopaminergic, glutamatergic, and GABAergic neurotransmission, mitochondrial dysfunction, oxidative stress, and neuroinflammation. While excessive levels of copper and iron can lead to the generation of reactive oxygen species by Fenton chemistry, manganese might increase reactive oxygen species production indirectly. Feeding rats a high manganese diet leads to an increase in markers of oxidative stress as well as a shift in the ratio Fe2+/Fe3+ in the brain [43]. This suggests that the change in Fe2+/Fe3+ might favor iron-induced production of reactive oxygen species.
Disorders Leading to Manganese Accumulation in the Brain
Manganese Poisoning
High levels of manganese in the blood can occur due to a high manganese intake, which is particularly likely if the mechanisms restricting entry through the gut are by-passed. Examples include parenteral nutrition, intravenous abuse of drugs contaminated with manganese, working in mines and battery factories, and welding [42].
Acquired Hepatocerebral Syndrome/Acquired Hepatocerebral Degeneration
In patients with chronic liver disease (particularly cirrhosis), manganese excretion is impaired and blood manganese rises with the deposition of manganese in the basal ganglia and subsequent motor impairment. Clinical characteristics include movement disorders, mainly parkinsonism and ataxia-plus syndrome, as well as cognitive impairment with psychiatric features. Neuroimaging studies of acquired hepatocerebral degeneration (AHD) with parkinsonism show hyperintensity in the bilateral globus pallidus on T1-weighted magnetic resonance images, consistent with manganese accumulation. Ataxia-plus syndrome in AHD may demonstrate high-signal lesions in the middle cerebellar peduncles on T2-weighted images [44]. Iron deficiency is common in patients showing brain MRI abnormalities compatible with manganese deposits in thr basal ganglia. This observation suggests that iron deficiency could be an important risk factor for manganese-induced neurotoxicity and should, therefore, be carefully considered and treated [45].
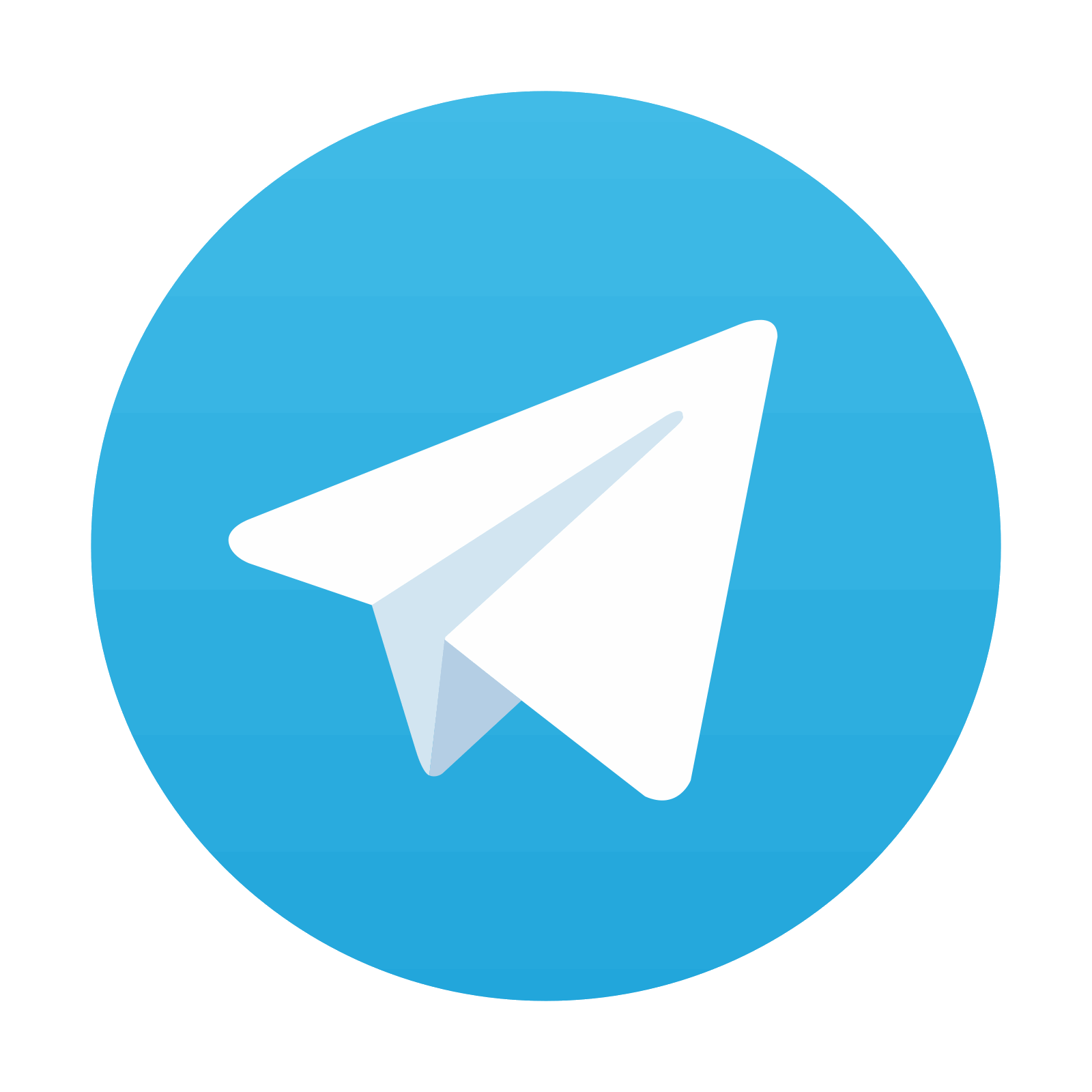
Stay updated, free articles. Join our Telegram channel
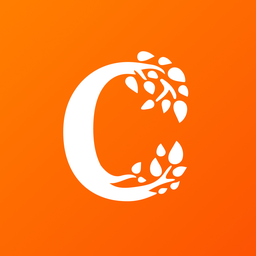
Full access? Get Clinical Tree
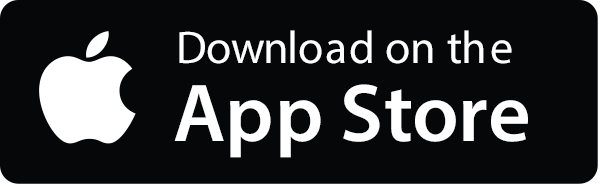
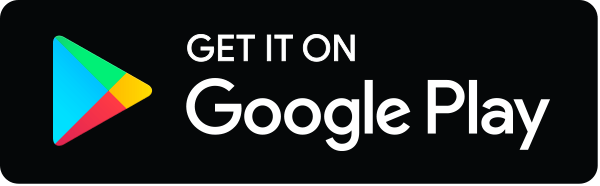
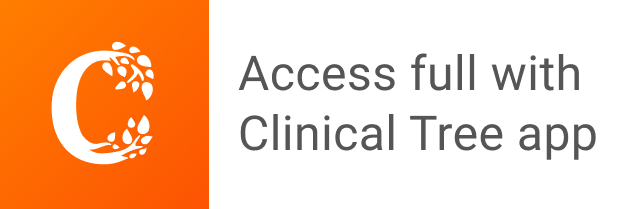