Normal Development and Deviations in Development of the Nervous System: Introduction
In this chapter and the next one on aging, we consider the effects of growth, maturation, and aging on the nervous system. These are discussed in some detail because certain aspects of neurologic diseases are meaningful only when viewed against the background of these natural age-linked changes. Developmental diseases of the nervous system, e.g., malformations, genetic defects, and other forms of damage that are acquired in the intrauterine period of life, are considered in Chap. 38.
Sequences of Normal Development
The establishment of a biologic timescale of human development requires observation of a large number of normal individuals of known ages and testing them for measurable items of behavior. Because of individual variations in the tempo of development, it is equally important to study the growth and development of any one individual over a prolonged period. If these observations are to be correlated with stages of neuroanatomic development, the clinical and morphologic data must be expressed in units that are comparable. Early in life, very precise age periods are difficult to ascertain because of the difficulty in fixing the time of conception. The average human gestational period is 40 weeks (280 days), but birth may occur with survival as early as about 24 or as late as 49 weeks (a time span of almost 5 months), and the extent of nervous system development varies accordingly.
After birth, any given item of behavior or structural differentiation must always have two reference points: (1) to a particular item of behavior that has already been achieved, and (2) to units of chronologic time or duration of life of the organism. The chronologic or biologic scale assumes special significance in early prenatal life. During that period development proceeds at such a rapid pace that small units of time weigh heavily and the organism appears to change literally day by day. In infancy, the tempo of development slows somewhat but is still very rapid in comparison with later childhood.
The neurologist will find it advantageous to organize knowledge of normal development and disease around the timetables for human growth and development listed in Tables 28-1 and 28-2. In addition, the last decade has brought startling advances in the understanding of the genetic and molecular control of neural development. That topic is considered in Chap. 38.
GROWTH PERIOD | APPROXIMATE AGE |
---|---|
Prenatal | From 0 to 280 days |
Ovum | From 0 to 14 days |
Embryo | From 14 days to 9 weeks |
Fetus | From 9 weeks to birth |
Premature infant | From 27 to 37 weeks |
Birth | Average 280 days |
Neonate | First 4 weeks after birth |
Infancy | First year |
Early childhood (preschool) | From 1 to 6 years |
Later childhood (prepubertal) | From 6 to 10 years |
Adolescence | Girls, 8 or 10 to 18 years |
Boys, 10 or 12 to 20 years | |
Puberty (average) | Girls, 13 years |
Boys, 15 years |
FETAL AGE, DAYS | SIZE (CROWN–RUMP LENGTH), MM | NERVOUS SYSTEM DEVELOPMENT |
---|---|---|
18 | 1.5 | Neural groove and tube |
21 | 3.0 | Optic vesicles |
26 | 3.0 | Closure of anterior neuropore |
27 | 3.3 | Closure of posterior neuropore; ventral horn cells appear |
31 | 4.3 | Anterior and posterior roots |
35 | 5.0 | Five cerebral vesicles |
42 | 13.0 | Primordium of cerebellum |
56 | 25.0 | Differentiation of cerebral cortex and meninges |
150 | 225.0 | Primary cerebral fissures appear |
180 | 230.0 | Secondary cerebral sulci and first myelination appear in brain |
8–9 months | 240.0 | Further myelination and growth of brain (see text) |
A large body of knowledge has accumulated concerning the functional and structural status of the nervous system during each successive period of life. This information is reviewed briefly in the following paragraphs and is summarized in Table 28-2. It must be kept in mind that development of the nervous system does not proceed stepwise, from one period to the next, but is continuous from conception to maturity. The sequences of development are much the same in all infants, although the rate may vary slightly. Any given behavioral function, in order to be expressed, must await the development of its neural substrate. Furthermore, at any given moment in development, several measurable functions appear in parallel, and it is often a dissociation between them that acquires clinical significance.
What we know of the nervous system in the germinal and embryonal periods has been derived from the study of a relatively small number of fetuses that have come into the hands of anatomists. Neuroblastic differentiation, migration, and neuronal multiplication are already well under way in the first 3 weeks of embryonic life. The control of each of these phases (and, later, of connectivity of neurons) is determined by the genome of the organism. Primitive cells destined to become neurons originate in, or close to, the neuroepithelium of the neural tube. These cells proliferate at an astonishingly rapid rate (250,000 per minute, according to Cowan) for a circumscribed period (several days to weeks). They become transformed into bipolar neuroblasts, which migrate in a series of waves toward the marginal layer of what is to become the cortex of the cerebral hemispheres. The first glial cells also appear very early and provide the scaffolding along which the neuroblasts move. Each step in the differentiation and migration of the neuroblasts proceeds in an orderly fashion, and one stage progresses to the next with remarkable precision. The process of neuronal migration is largely completed by the end of the fifth fetal month but continues at a much slower rate up to 40 weeks of gestation, according to the classic studies of Conel and of Rabinowicz. Because the migration of most neurons involves postmitotic cells, the cerebral cortex by this time has presumably acquired its full complement of nerve cells, numbered in the many billions. This concept has been revised in recent years with the discovery that active stem cells in the adult brain generate neurons in the hippocampal formation and in the subventricular matrix zones, giving rise most evidently to olfactory neurons in the adult brain but possibly also to other nerve cells (see Kempermann and also Alvarez-Buylla and Garcia-Verdugo).
Despite the almost universal acceptance of the presence of neuron genesis in the human brain, the methodology that demonstrates it is complex and has been questioned by authoritative individuals such as Rakic, whose perspective should be consulted for a complete portrait of the subject. Actually, we have little idea of the number of nerve cells in the cerebral and cerebellar cortices at different ages. Many more are formed than survive, as programmed cell death (apoptosis) constitutes an important component of development.
Within a few months of mid-fetal life, the cerebrum, which begins as a small bihemispheric organ with hardly a trace of surface indentation, evolves into a deeply sulcated structure. Every step in the folding of the surface to form fissures and sulci follows a temporal pattern of such precision as to permit a reasonably accurate estimation of fetal age by this criterion alone. The major sylvian, rolandic, and calcarine fissures take on the adult configuration during the fifth month of fetal life, the secondary sulci in the sixth and seventh months, and the tertiary sulci, which vary slightly in location from one individual to another, in the eighth and ninth months (see Fig. 28-1 and Table 28-2).
Concomitantly, subtle changes in neuronal organization are occurring in the cerebral cortex and central ganglionic masses. Involved here are the processes of synaptogenesis and axonal pathfinding. Neurons become more widely separated as differentiation proceeds, owing to an increase in the size and complexity of dendrites and axons and enlargement of synaptic surfaces (Fig. 28-2).
The cytoarchitectural patterns that demarcate one part of the cerebral cortex from another (as described in Chap. 22) are already in evidence by the thirtieth week of fetal life and become definitive at 40 weeks and in succeeding months. As the maturational process of cortical neurons proceeds, the patterns of neuronal organization in different regions of the brain (motor, premotor, sensory, and striate cortices, Broca and Wernicke areas) continue to change.
Figure 28-2.
Cox-Golgi preparations of the leg area of the motor cortex (area 4). Upper row, left to right: 1 month premature (8 months gestation); newborn at term; 1 month; 3 months; and 6 months. Lower row, left to right: 15 months; 2 years; 4 years; 6 years. Apical dendrites of Betz cells have been shortened, all to the same degree, for the purposes of display. (Courtesy of T. Rabinowicz, University of Lausanne.)
Myelination provides another parallel index of development and maturation of the nervous system and is apparently related to the functional activity of the fiber systems. The timing and precision of these connecting pathways are no less precise and time locked than is neuronal development (see Flechsig’s myelinogenic cycle as shown in Fig. 28-3). The acquisition of myelin sheaths by the spinal nerves and roots by the tenth week of fetal life is associated with the beginning of reflex motor activities. Segmental and intersegmental fiber systems in the spinal cord myelinate soon afterward, followed by ascending and descending fibers to and from the brainstem (reticulospinal, vestibulospinal). The acoustic and labyrinthine systems stand out with singular clarity in myelin-stained preparations by the twenty-eighth to thirtieth weeks, and the spinocerebellar and dentatorubral systems by the thirty-seventh week.
After birth, the brain continues to grow dramatically. From an average weight of 375 to 400 g at birth (40 weeks), it reaches about 1,000 g by the end of the first postnatal year. Glial cells (oligodendrocytes and astrocytes) derived from the matrix zones continue to divide and multiply during the first 6 months of postnatal life. The visual system begins to myelinate about the fortieth gestational week; its myelination cycle proceeds rapidly, being nearly complete a few months after birth. The corticospinal tracts are not fully myelinated until halfway through the second postnatal year. Most of the principal tracts are myelinated by the end of this period. In the cerebrum, the first myelin is seen at 40 weeks in the posterior frontal and parietal lobes, and the occipital lobes (geniculocalcarine tracts) myelinate soon thereafter. Myelination of the anterior frontal and temporal lobes occurs later, during the first year of postnatal life. By the end of the second year, myelination of the cerebrum is largely complete (see Fig. 28-3). These steps in myelination can be followed by MRI. Despite these careful anatomic observations, their correlation with developmental clinical and electroencephalographic data has not been precise.
Growth of the brain continues, at a much slower rate than before, until 12 to 15 years, when the average adult weight of 1,230 to 1,275 g in females and 1,350 to 1,410 g in males is attained. Myelination also continues slowly during this period. Yakovlev and Lecours, who reexamined Flechsig’s classic findings on the ontogeny of myelination (the term Flechsig’s myelinogenic cycle is still used), traced the progressive myelination of the middle cerebellar peduncle, acoustic radiation, and bundle of Vicq d’Azyr (mammillothalamic tract) beyond the third postnatal year; the nonspecific thalamic radiations continued to myelinate beyond the seventh year and fibers of the reticular formation, great cerebral commissures, and intracortical association neurons to at least the tenth year and beyond (see Fig. 28-3). These investigators noted that there was an increasing complexity of fiber systems through late childhood and adolescence, and perhaps even into middle adult life. Similarly, in the extensive studies of Conel and Rabinowicz, depicting the cortical architecture at each year from mid-fetal life to the twentieth year, the dendritic arborizations and cortical interneuronal connections were observed to increase progressively in complexity; the “packing density” of neurons, i.e., the number of neurons in any given volume of tissue increases through the age of approximately 15 months and then decreases (see Fig. 28-2).
Interesting questions are whether neurons begin to function only when their axons have acquired a myelin sheath; whether myelination is under the control of the cell body, the axon, or both; and whether the usual myelin stains yield sufficient information as to the time of onset and degree of the myelination process. At best these correlations can be only approximate. It seems likely that systems of neurons begin to function before the first appearance of myelin, at least insofar as shown in conventional myelin stains. These correlations will undoubtedly be restudied using more delicate measures of function and finer staining techniques, as well as the techniques of quantitative biochemistry and phase and electron microscopy.
The human fetus is capable of a complex series of reflex activities, some of which appear as early as 5 weeks of postconceptional age. Cutaneous and proprioceptive stimuli evoke slow, generalized, patterned movements of the head, trunk, and extremities. More discrete movements appear to differentiate from these generalized activities. Reflexes subserving blinking, sucking, grasping, and visceral functions, as well as tendon and plantar reflexes, are all elicitable in late fetal life. They seem to develop along with the myelination of peripheral nerves, spinal roots, spinal cord, and brainstem. By the twenty-fourth week of gestation, the neural apparatus is functioning sufficiently well to give the fetus some chance of survival should birth occur at this time. However, most infants fail to survive birth at this age, usually owing to an inadequacy of pulmonary function. Thereafter, the basic neural equipment matures so rapidly that, by the thirtieth week, postnatal viability is relatively common. It seems that nature prepares the fetus for the contingency of premature birth by hastening the establishment of vital functions necessary for extrauterine existence.
It is in the last trimester of pregnancy that a complete timetable of fetal movements, posture, and reflexes would be of the greatest value, for mainly during this period does the need for a full clinical evaluation arise. That there are recognizable differences between infants born in the sixth, seventh, eighth, and ninth months of fetal life has been documented by Saint-Anne Dargassies, who applied the neurologic tests earlier devised by André-Thomas and herself. Her observations document prevailing postures; control and attitude of head, neck, and limbs; muscle tonus; and grasp and sucking reflexes. These findings are of interest and may well be a means of determining exact age, but many more observations are needed with follow-up data on later development before they can be fully accepted. Part of the difficulty here is the variability of the premature infant’s neurologic functions, which may change literally from hour to hour. Even at term there may be variability in neurologic functions from one day to the next. This variability reflects in part the effects of parturition and the effects of drugs given to the mother, as well as the inaccurate dating of conception and rapid developmental changes in the brain.
At term, effective sucking, rooting, and grasping reactions are present. The infant is able to swallow and cry, and the startle reaction (e.g., Moro reflex, as described further on) can be evoked by loud sound and sudden extension of the neck. Support and steppage movements can be demonstrated by placing the infant on its feet, and incurvation of the trunk by stroking one side of the back. Also present at birth is the placing reaction, wherein the foot or hand, brought passively into contact with the edge of a table, is lifted automatically and placed on the flat surface. These neonatal automatisms depend essentially on the functioning of the spinal cord, brainstem, and possibly diencephalon and pallidum. The Apgar score, a universally used but somewhat imprecise index of the well-being of the newly born infant, is in reality a numerical rating of the adequacy of brainstem-spinal mechanisms (breathing, pulse, color of skin, tone, and responsivity) (Table 28-3).
Heart rate |
0 No heart rate |
1 Fewer than 100 beats per minute—the baby is not very responsive |
2 More than 100 beats per minute—the baby is obviously vigorous |
Respiration |
0 Not breathing |
1 Weak cry; may sound like wimpering or grunting |
2 Good strong cry |
Muscle tone |
0 Limp |
1 Some flexing (bending) of arms and legs |
2 Active motion |
Reflex response |
0 No response to airways being suctioned |
1 Grimace during suctioning |
2 Grimace and cough or sneeze during suctioning |
Color |
0 The baby’s whole body is completely blue or pale |
1 Good color in body with blue hands or feet |
2 Completely pink or good color |
Studies of local cerebral glucose metabolism by positron emission tomography (PET) have provided interesting information about the functional maturation of the brain. There are remarkable differences between the newborn and the mature individual. Neonatal values, adjusted for brain weight, are only one-third those of the adult; except for the primary sensorimotor cortex, they are confined to brainstem, cerebellum, and thalamus. During infancy, there is a progressive evolution in the pattern of glucose metabolism in the parietal, temporal, striate, dorsolateral occipital, and frontal cortices, in this order. Only by the end of the first year do the glucose metabolic patterns qualitatively resemble those of the normal young adult (Chugani).
Behavior during infancy and early childhood is also the subject of a substantial literature, contributed more by psychologists than neurologists. In particular they have explored sensorimotor performance in the first year and language and social development in early childhood. In the first 6 years of life, the infant and young child traverse far more ground developmentally than they ever will again in a similar period. From the newborn state, when the infant demonstrates a few primitive feeding and postural reflexes, there are acquired, within a few months, smiling and head and hand–eye control; by 6 months, the ability to sit; by 10 months, the strength to stand; by 12 months, the muscle coordination required to walk; by 2 years, the ability to run; and by 6 years, mastery of the rudiments of a game of baseball or a musical skill. On the perceptual side, the neonate progresses, in less than 3 months, from a state in which ocular control is tentative and tonic deviation of the eyes occurs only in response to labyrinthine stimulation to one in which he is able to fixate on and follow an object. (This last corresponds to the development of the macula.) Later, the child is able to make fine discriminations of color, form, and size. Gesell has provided a graphic summation of the variety and developmental sweep of a child’s behavior. He writes
At birth the child reflexly grasps the examiner’s finger, with eyes crudely wandering or vacantly transfixed . . and by the sixth year the child adaptively scans the perimeter of a square or triangle, reproducing each form with directed crayon. The birth cry, scant in modulation and social meaning, marks the low level of language, which in two years passes from babbling to word formation that soon is integrated into sentence structure, and in six years to elaborated syntactic speech with questions and even primitive ideas of causality. In personality makeup . . the school beginner is already so highly organized, both socially and biologically, that he foreshadows the sort of individual he will be in later years.
The studies of Gesell and Amatruda and of others represent attempts to establish age-linked standards of behavioral development, but the difficulties of using such rating scales are considerable. The components of behavior that have been chosen as a frame of reference for neurological development are not likely to be of uniform physiologic value or of comparable complexity, and they have seldom been standardized on large populations drawn from different cultures. Also, the examinations at specified ages are cross-sectional assessments, which give a limited idea of the dynamics of behavioral development. As already stated, temporal patterns of behavior reveal an extraordinary degree of variation in their emergence, increment, and decrement, as well as marked variation from one individual to another.
Indeed, the predictive value of developmental assessment has been the subject of a lively dispute. Gesell took the position that careful observation of a large number of infants, with accurate recording of the age at which various skills are acquired, permits the establishment of norms or averages. From such a framework one can determine the level of developmental attainment, expressed as the development quotient (DQ = developmental age/chronologic age), and thus ascertain whether any given child has superior, average, or inferior performance. Furthermore, after examining 10,000 infants over a period of 40 years, Gesell concluded that “attained growth is an indicator of past growth processes and a foreteller of growth yet to be achieved.” In other words, the DQ predicts potential attainment.
The other position—taken by Anderson and others—is that developmental attainments are of no real value in predicting the level of intelligence but are measures of completely different functions. Illingworth and most clinicians, including the authors, have taken an intermediate position, that the developmental scale in early life is a useful source of information, but it must be combined with a full clinical assessment. When this is done, the clinician has a reasonably certain means of detecting delays in cognitive development and other forms of neurologic impairment.
The trajectory of rapid growth and maturation continues in late childhood and adolescence, although at a slower pace than before. Motor skills attain their maximal precision in the performances of athletes, artists, and musicians, whose peak development is at maturity (age 18 to 21 years). Intelligence and the capacity for reflective thought and the manipulation of mathematical symbols become possible for most individuals only in adolescence and later. Emotional control, precarious in the school age and all through adolescence, stabilizes in adulthood. We tend to think of all these phenomena as being achieved through the stresses of human relations, which are conditioned and habituated by the powerful influences of social approval. In this extensive and pervasive interaction between the individual and the environment, which is the preoccupation of the child psychiatrist, it is well to remember that the processes of extrinsic and intrinsic organization can be separated only for the purpose of analytic discussion. There is always interdependence between them.
As indicated earlier and in Table 28-4, the wide variety and seemingly random movements displayed by the healthy neonate are from birth, and certainly within days, firmly organized into reflexive-instinctual patterns called automatisms. The most testable of the automatisms are blinking in response to light, tonic deviation of the eyes in response to labyrinthine stimulation (turning of the head), prehensile and sucking movements of the lips in response to labial contact, swallowing, avoidance movements of the head and neck, startle reaction (Moro response, see further on) in response to loud noise or dropping of the head into an extended position, grasp reflexes, and support, stepping, and placing movements. This repertoire of movements, as mentioned earlier, depends on reflexes organized mainly at the spinal and brainstem levels. Only the placing reactions, ocular fixation, and following movements (the latter are established by the third month) are thought to depend on emerging cortical connections, but even this is debatable. In the neonatal period, when little of the cerebrum has begun to function, extensive cerebral lesions may cause little derangement of motor function and may pass unnoticed unless special methods of examination—sensory evoked potentials, electroencephalography (EEG), CT, and MRI—are used. Of clinically testable neurologic phenomena in the neonatal period, disturbances of ocular movement, seizures, tremulousness of the arms, impaired arousal reactions and muscular tone, all of which relate essentially to upper brainstem and diencephalic mechanisms, provide the most reliable clues to the presence of neurologic disease. Prechtl and associates have affirmed the importance of disturbances of these neurologic functions at this early age as predictors of delayed development.
AGE | NORMAL FUNCTIONS | PATHOLOGIC SIGNS |
---|---|---|
Newborn period | Blinking, tonic deviation of eyes on turning head, sucking, rooting, swallowing, yawning, grasping, brief extension of neck in prone position, incurvation response, Moro response, flexion postures of limbs | Lack of arousal (stupor or coma) |
High-pitched or weak cry | ||
Abnormal (incomplete or absent) Moro response | ||
Opisthotonus | ||
Biceps reflexes present and others variable; infantile type of flexor plantar reflex; stable temperature, respirations, and blood pressure; periods of sleep and arousal: vigorous cry | Flaccidity or hypertonia | |
Convulsions | ||
Tremulous limbs | ||
Failure of tonic deviation of eyes on passive movement of head or of head and body | ||
2–3 months | Supports head | Absence of any or all of the normal functions |
Smiles | Convulsions | |
Makes vowel sounds | Hypotonia or hypertonia of neck and limbs | |
Adopts tonic asymmetrical neck postures (tonic neck reflexes) | Vertical suspension—legs extend and adduct | |
Large range of movements of limbs, tendon reflexes usually present | ||
Fixates on and follows a dangling toy | ||
Suckles vigorously | ||
Period of sleep sharply differentiated from awake periods | ||
Support and stepping unelicitable | ||
Vertical suspension—legs flex, head up | ||
Optokinetic nystagmus elicitable | ||
4 months | Good head support, minimal head lag | Lack of head support |
Coos and chuckles | Motor deficits | |
Inspects hands | Hypertonia | |
Tone of limbs moderate or diminished | Lack of social reactions | |
Turns to sounds | Tonic neck reflexes present | |
Rolls over from prone to supine | Strong Moro response | |
Grasping, sucking, and tonic neck reflexes subservient to volition | Absence of symmetrical attitude | |
5–6 months | Babbles | Altered tone |
Reaches and grasps | Obligatory postures | |
Vocalizes in social play | Cannot sit or roll over | |
Discriminates between family and strangers | Hypo- or hypertonia | |
Moro and grasp reflexes disappear | Persistent Moro and grasp | |
Tries to recover lost object | Persistent tonic neck reflexes | |
Begins to sit; no head lag on pull to sit | No Landau response | |
Positive support reaction | ||
Tonic neck reflexes gone | ||
Landau response (holds head above horizontal, arches back when held horizon-tally) | ||
Begins to grasp objects with one hand; holds bottle | ||
9 months | Creeps and pulls to stand; stands holding on | Fails to attain these motor, verbal, and social milestones |
Sits securely | Persistent automatisms and tonic neck reflexes or hypo- or hypertonia | |
Babbles “Mama,” “Dada,” or equivalent | ||
Sociable; plays “pat-a-cake,” seeks attention | ||
Drinks from cup | ||
Landau response present | ||
Parachute response present | ||
Grasps with thumb to forefinger | ||
12 months | Stands alone | Failure to attain 12-month milestones |
May walk, or walks if led | Persistence of automatisms | |
Tries to feed self | ||
May say several single words, echoes sounds | ||
Plantar reflexes definitely flexor | ||
Throws objects | ||
15 months | Walks independently (9–16 months), falls easily | Retardation in reaching milestones expected at this age |
Moves arms steadily | Persistent abnormalities of tone and posture | |
Says several words; scribbles with crayon | Sensory discriminations defective | |
Requests by pointing | ||
Interest in sounds, music, pictures, and animal toys | ||
18 months | Says at least 6 words | Cannot walk |
Feeds self; uses spoon well | No words | |
May obey commands | ||
Runs stiffly; seats self in chair | ||
Hand dominance | ||
Throws ball | ||
Plays several nursery games | ||
Uses simple tools in imitation | ||
Removes shoes and stockings | ||
Points to two or three parts of body, common objects, and pictures in book | ||
24 months | Says 2- or 3-word sentences Scribbles Runs well; climbs stairs one at a time Bends over and picks up objects Kicks ball; turns knob Organized play Builds tower of 6 blocks Sometimes toilet trained | Retarded in all motor, linguistic, and social adaptive skills |
During early infancy, the motor system undergoes a variety of differentiations as visual, auditory, and tactile motor mechanisms develop. Bodily postures are modified to accommodate these complex sensorimotor acquisitions. In the normal infant, these emerging motor differentiations follow a time schedule prescribed by the maturation of neural connections. Normalcy is expressed by the age at which each of these appears, as shown in Table 28-4. It is also evident from this table that reflex and instinctual motor activities are the most dependable means of evaluating early development. Moreover, in the normally developing infant, some of these activities disappear as others appear. For example, the grasp reflex, extension of the limbs without a flexor phase, Moro response, tonic neck reflexes, and crossed adduction in response to eliciting the knee jerk gradually become less prominent and are usually not elicitable by the sixth month. The absence of these reflexes in the first few months of life and conversely, their persistence beyond this time indicate a defect in cerebral development, as described in more detail further on, under “Delays in Motor Development.” By contrast, neck-righting reflexes, support reactions, the Landau reaction (extending neck and legs when held prone), the parachute maneuver, and the pincer grasp, which are absent in the first 6 months, begin to appear by the seventh to eighth months and are present in all normal infants by the twelfth month.
Because many functions that are classified as “mental” at a later period of life have a different anatomic basis than motor functions, it is not surprising that early motor achievements do not correlate closely with childhood intelligence. The converse does not apply, however; delay in the acquisition of motor milestones often correlates with developmental delay. In other words, most cognitively delayed children sit, stand, walk, and run at a later age than normal children, and deviations from this rule occur mainly in special diseases such as autism.
In the period of early childhood, the reflexive-instinctual activities are no longer of help in evaluating cerebral development, and one must turn to the examination of language functions and learned sensory and motor skills, which are outlined in Tables 28-5 and 28-6.
AGE | OBSERVED ITEMS | USEFUL CLINICAL TESTS |
---|---|---|
2 years | Runs well | Pencil-paper test: scribbles, imitates horizontal stroke |
Goes up and down stairs, 1 step at a time | Folds paper once | |
Climbs on furniture | Builds tower of 6 blocks | |
Opens doors | ||
Helps to undress | ||
Feeds well with spoon | ||
Puts 3 words together | ||
Listens to stories with pictures | ||
2.5 years | Jumps on both feet; walks on tiptoes if asked | Pencil-paper test: copies horizontal and vertical line |
Knows full name; asks questions | Builds tower of 8 blocks | |
Refers to self as “I” | ||
Helps put away toys and clothes | ||
Names animals in book, knows 1 to 3 colors | ||
Can complete 3-piece form board | ||
3 years | Climbs stairs, alternating feet | Builds 9-cube tower |
Talks constantly; recites nursery rhymes | Builds bridge with 3 cubes | |
Rides tricycle | Imitates circle and cross with pencil | |
Stands on one foot momentarily | ||
Plays simple games | ||
Helps in dressing | ||
Washes hands | ||
Identifies 5 colors | ||
4 years | Climbs well; hops and skips on one foot; throws ball overhand; kicks ball | Copies cross and circle |
Builds gate with 5 cubes | ||
Cuts out pictures with scissors | Builds a bridge from model | |
Counts 4 pennies | Draws a human figure with 2 to 4 parts other than head | |
Tells a story; plays with other children | Distinguishes short and long line | |
Goes to toilet alone | ||
5 years | Skips | Copies square and triangle |
Names 4 colors; counts 10 pennies | Distinguishes heavier of 2 weights | |
Dresses and undresses | More detailed drawing of a human figure | |
Asks questions about meaning of words |
DEFICIT | TEST |
---|---|
Development | Denver Developmental Test; Vineland Social Maturity Test; Leiter International Performance Scale; Otis Group Intelligence Test |
Achievement | Wide Range Achievement Test; Gates Primary Reading Test |
Attentiveness | Dehoit Test of hearing aptitude |
Calculation | Key Math Diagnostic Arithmetic Test |
Vocabulary | Peabody Picture Vocabulary |
Developmental Gerstmann syndrome (finger agnosia, right–left disorientation) | Finger Order Tests; Benton Right–Left Discrimination Test |
Figure copying | Visual–Motor Integration Test |
Visual memory | Benton Visual Retention Test |
Error patterns | Boder Test of Reading–Spelling Patterns |
Impulsiveness | Matching Familiar Figures Test |
Quite apart from the early stage of motor development, in later childhood and adolescence one observes a remarkable variation in levels of muscular activity, strength, and coordination. Motor acquisitions of later childhood such as hopping on one foot, kicking a ball, jumping over a line, walking gracefully, dancing, and certain skills in sports are linked to age but there is wide variability in the finesse with which they are performed. Ozeretzkii has combined these in a scale that putatively discloses arrests in motor development in the developmentally delayed. Also in later childhood, precocity in learning complex motor skills as well as skill in games and the development of an all-around interest in athletic activity becomes evident. By adolescence, high individual physical achievement is well recognized. At the other end of the spectrum are instances of motor underachievement, ineptitude, and intrinsic awkwardness; a member of this group will easily stand out and be designated as “an awkward child.” Such awkwardness is to be clearly distinguished from the motor impairment associated with a number of cerebral diseases.
Under normal circumstances, sensory development keeps pace with motor development, and at every age sensorimotor interactions are apparent. However, under conditions of disease, this generalization may not hold; i.e., motor development may remain relatively normal in the face of a sensory defect, or vice versa. The sense organs are fully formed at birth. The neonate is crudely aware of visual, auditory, tactile, and olfactory stimuli, which elicit only low-level reflex responses. Moreover, any stimulus-related response is only to the immediate situation; there is no evidence that previous experience with the stimulus has influenced the response; i.e., that the newborn can learn and remember. The capacity to attend to a stimulus, to fixate on it for any period of time, also comes later. Indeed, the length of fixation time is a quantifiable index of perceptual development in infancy.
Information is available about the time at which the infant makes the first interpretable responses to each of the different modes of stimulation. The most nearly perfect senses in the newborn are those of touch and pain. A series of pinpricks cause distress, whereas an abrasion of the skin seems not to do so. The sense of touch clearly plays a role in feeding behavior. Newborn infants react vigorously to irritating odors such as ammonia and acetic acid, but discrimination between olfactory stimuli is not evident until much later. Sugar solutions initiate and maintain sucking from birth on, whereas quinine (bitter) solutions seldom do, and the latter stimulus elicits avoidance behavior. Hearing in the newborn is manifest within the first few postnatal days. Sharp, quick sounds elicit responsive blinking and sometimes startle. In some infants, the human voice appears to cause similar reactions by the second week. Strong light and objects held before the face evoke reactions in the neonate; later, visual searching is an integrating factor in most projected motor activities.
Sensation in the newborn infant must be judged largely by its motor reactions, so that sensory and motor developments seem to run in parallel but this may be partly artifactual. There are nonetheless discernible maturational stages that constitute sensory milestones, so to speak. This is most apparent in the visual system, which is more easily studied than the other senses. Sustained ocular fixation on an object is observable at term and even in some preterm infants; at these ages it is essentially a reflexive phototropic reaction. It has been observed that the neonate will consistently gaze at some stimuli more often than others, suggesting, according to Fantz, that there must already be some elements of perception and differentiation at this early stage. Voluntary fixation (i.e., following a moving object) is a later development. Horizontal following occurs at about 50 days; vertical following, at 55 days; and following an object that is moving in a circle, at 75 days. Preference for a colored stimulus over a gray one was recorded by Staples by the end of the third month. By 6 months the infant discriminates between colors, and saturated colors can be matched at 30 months. Perception of form, at least as judged by the length of time spent in looking at different visual presentations, is evident at 2 or 3 months of age (Fantz). At this time infants are attracted more to certain patterns than to colors. At 3 months, most infants have discovered their hands and spend considerable time watching their movements. The ages at which infants begin to observe color, size, shape, and numbers can be determined by means of the Terman-Merrill and Stutzman intelligence tests (see Gibson and Olum). Perception of size becomes increasingly accurate in the preschool years. An 18-month-old child discriminates among pictures of familiar animals and recognizes them equally well if they are upside down.
Visual discrimination is reflected in manual reactions, just as auditory discrimination is reflected in vocal responses. Much of early visual development (first year) involves peering at objects, judging their position, reaching for them, and seizing and manipulating them. The inseparability of sensory and motor functions is never more obvious. Sensory deprivation impedes not only the natural sequences of perceptual awareness of the child’s surroundings but also the development of all motor activities. Auditory discrimination—reflected in vocalizations such as babbling and, later, in word formation—is discussed further on in connection with language development.
The subject of intellectual endowment and the development and testing of intelligence were touched upon in Chap. 21. There it was pointed out that although intelligence is modifiable by training, practice, and schooling, it is much more a matter of native endowment and not simply a question of environment and providing the stimulus to learn, although these are clearly factors. It is evident early in life that some individuals have a superior intelligence; they also clearly maintain this differentiation all through life, and the opposite pertains in others.
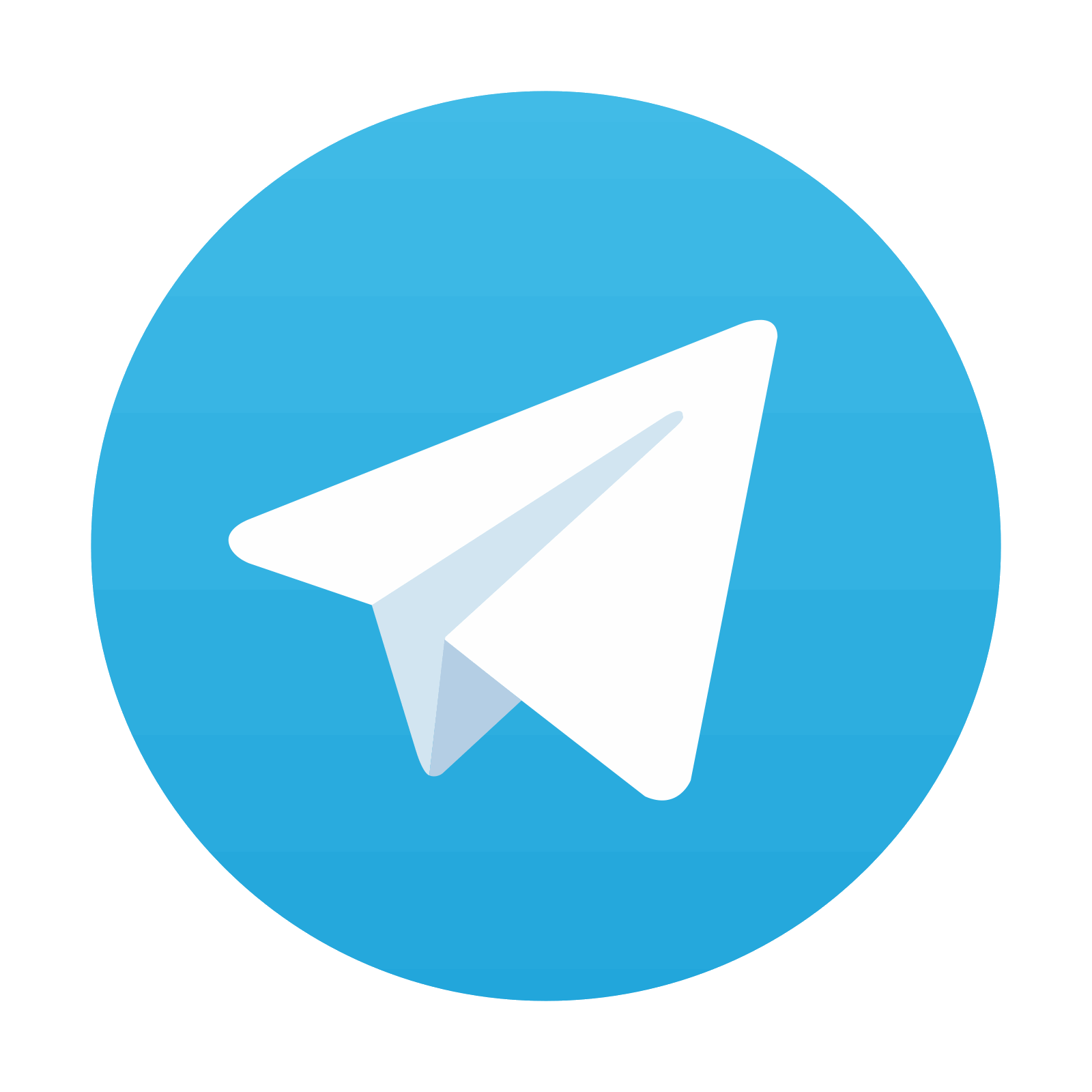
Stay updated, free articles. Join our Telegram channel
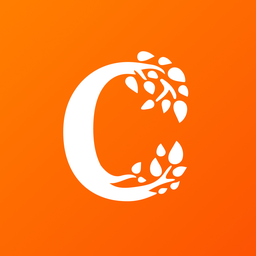
Full access? Get Clinical Tree
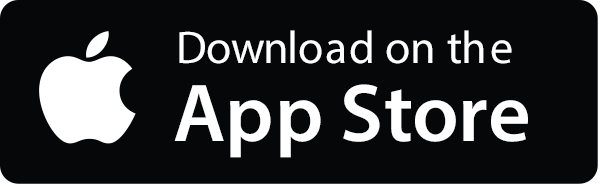
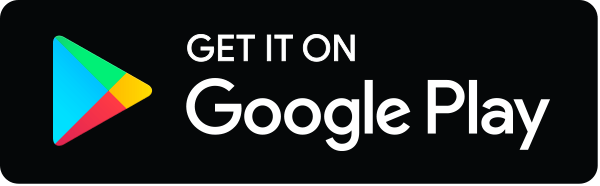