Abstract
Hereditary spastic paraplegias (HSPs) represent a large and heterogeneous group of inherited disorders, presenting with a phenotype that is predominated by lower limb spasticity and weakness, often accompanied by pyramidal-tract signs and neurogenic bladder dysfunction. This phenotype is typically associated with the degeneration of the corticospinal tract that leads to the hallmark manifestations of the condition. The HSPs have been traditionally divided into pure and complicated forms.
Introduction
Hereditary spastic paraplegias (HSPs) represent a large and heterogeneous group of inherited disorders, presenting with a phenotype that is predominated by lower limb spasticity and weakness, often accompanied by pyramidal-tract signs and neurogenic bladder dysfunction. This phenotype is typically associated with the degeneration of the corticospinal tract that leads to the hallmark manifestations of the condition. The HSPs have been traditionally divided into pure and complicated forms. Patients with pure HSP present isolated pyramidal signs and mild sensory symptoms with predominantly diminished vibration sensation, while patients with complicated HSP have other accompanying neurological or systemic signs, such as cognitive impairment, epilepsy, ataxia, extrapyramidal movement disorders, dysmorphic features, and peripheral neuropathy among others [1, 2].
The genetic and molecular background of HSPs is complex. More than 70 associated loci and more than 60 different genes have been identified to date, including all types of Mendelian inheritance, such as autosomal-dominant (AD), autosomal-recessive (AR), and X-linked forms [3, 4]. Several molecular pathways are involved in the pathogenesis of HSPs: membrane trafficking, mitochondrial function, myelination, cytoskeleton stability, autophagy, and protein or RNA metabolism [5, 6].
The same syndromic phenotype of predominantly lower limb spasticity and weakness can be caused by other inherited or acquired neurological conditions, including motor neuron disorders, spinocerebellar ataxia, various forms of myelopathies, neurodegenerative disorders, as well as inherited leukodystrophies and other inborn errors of metabolism (IEMs) [7, 8].
The IEMs are a continuously expanding group of monogenic inherited metabolic disorders, today representing more than 750 different conditions, most of which are rare but together have an estimated prevalence of more than 1 in 1,000 live births. IEMs are generally caused by specific defects in various enzymes, cofactors, and transporters involved in specific cellular pathways. The presenting symptoms may result from enzyme deficiencies, from the accumulation of metabolic products that have toxic effects on tissues and organs, or from both. Inheritance of IEMs usually follows an autosomal-recessive pattern, but some conditions are inherited in an autosomal-dominant or X-linked fashion, and sporadic cases exist as well [9, 10].
Neurological symptoms are not uncommon in IEMs and range from severe encephalopathy, accompanied by hypotonia and seizures in neonates, to clinical heterogeneous and sometimes attenuated forms that present with a later onset during childhood, adolescence, or adulthood. The presenting symptoms and signs encountered may vary significantly and include spasticity, cognitive decline, cerebellar ataxia, extrapyramidal syndromes, seizures, psychiatric manifestations, or peripheral neuropathy [9, 10].
Motor neurons represent a significant target of the metabolic defects caused by IEMs. Therefore pyramidal symptoms and signs, including lower limb spasticity and weakness, are often present and occasionally produce a phenotype that resembles pure or complicated HSPs. The particular vulnerability of pyramidal cells might be explained by their long axons with high energy and transport demand [11].
Since most of the associated clinical syndromes have an onset during infancy and childhood, IEMs deserve a significant consideration in the differential diagnosis of childhood-onset HSPs [12]. It is not rare, though, that even adult cases with an HSP phenotype can be caused by various IEMs, either due to later onset of disease, or a milder phenotype that may result in diagnostic delay [11, 13].
Here, we summarize and review the most common IEMs that are associated with prominent lower limb spasticity and weakness and various pyramidal signs (Table 29.1). The classification of IEMs is done according to the latest proposed scheme by the Society for the Study of Inborn Errors of Metabolism, and includes disorders of intermediary metabolism, such as cofactor- and amino acid-related disorders, and disorders of complex molecule metabolism, such as peroxisomal and lysosomal storage disorders that cause various types of leukodystrophies [9, 10]. Many of these conditions are covered in detail in other chapters.
Table 29.1 Classification and main characteristics of IEMs presenting with phenotypes that resemble HSPs
Gene | Pattern of inheritance* | Age of onset | Common additional clinical features | |
---|---|---|---|---|
Cofactor-related disorders | ||||
Homocysteine remethylation defects |
|
|
|
|
Biotinidase deficiency | BTD | AR | Childhood to adulthood | Optic atrophy, ataxia, hearing defects, respiratory problems, skin manifestations |
Urea cycle disorders | ||||
Arginase deficiency | ARG1 | AR | Infancy to early childhood (rarely adolescence/adulthood) | Cognitive decline, epilepsy, cerebellar ataxia, dystonia, extrapyramidal signs |
HHH syndrome | SLC25A1S | AR | Infancy to adulthood | Learning disabilities, ataxia, epileptic seizures, episodes of confusion |
Disorders of phenylalanine metabolism | ||||
Phenylketonuria | PAH | AR | Infancy to childhood | Cognitive decline, visual disturbances, cerebellar ataxia, parkinsonism |
Dopamine synthesis defects | ||||
Atypical dopa-responsive dystonia (DRD-plus) |
|
| Childhood to adulthood | Dystonia, axial hypotonia, oculogyric crises, cerebellar ataxia, cognitive decline |
Organic acidurias | ||||
3-Methylglutaconic aciduria type 3 | OPA3 | AR | Infancy to early childhood | Optic atrophy, choreoathetosis, ataxia, mild cognitive deficits |
Peroxisomal disorders | ||||
Adrenomyeloneuropathy | ABCD1 | X-linked | Adulthood | Peripheral neuropathy |
Lysosomal disorders | ||||
Metachromatic leukodystrophy | ARSA | AR | Late infancy to adulthood | Cognitive decline, psychiatric symptoms, ataxia, peripheral neuropathy |
Krabbe disease | GALC | AR | Childhood to adulthood (rarely) | Cognitive decline, ataxia, optic atrophy, peripheral neuropathy |
Disorders of lipid and lipoprotein metabolism | ||||
Sjögren–Larsson syndrome | ALDH3A2 | AR | Childhood | Cognitive impairment, reduced visual acuity/photophobia, congenital ichthyosis |
Disorders of bile acid biosynthesis | ||||
Cerebrotendinous xanthomatosis | CYP27A1 | AR | Childhood to adulthood | Cognitive decline, cerebellar ataxia, dystonia/parkinsonism, seizures, peripheral neuropathy, tendon xanthomas, early-onset cataracts |
Congenital disorders of glycosylation | ||||
Adult polyglucosan body disease | GBE1 | AR | Late adulthood | Peripheral neuropathy |
* AD, autosomal-dominant; AR, autosomal-recessive.
It is important to note that several of spastic paraplegia (SPG)-designated genes have an important association with metabolic defects, including impaired amino acid metabolism, defective fatty acid and phospholipid metabolism, and congenital defects of autophagy, representing an emerging subclass of inherited errors of metabolism. It is beyond the scope of this chapter to present them in detail, but the relevant genes and conditions are summarized in Table 29.2.
Gene | Pattern of inheritance* | Metabolic defect | Phenotype | |
---|---|---|---|---|
SPG5A | CYP7B1 | AR | Defect in the degradation of cholesterol to primary bile acids | Pure HSP, or complicated HSP with presence of cerebellar signs, extrapyramidal signs, and peripheral neuropathy |
SPG9 | ALDH18A1 | AD or AR | Defect in the biosynthesis of ornithine and proline | Complicated HSP with cataracts, motor neuronopathy, ataxia, and cognitive impairment |
SPG11 | SPG11 | AR | Defect of autophagy | Complicated HSP with cognitive impairment, peripheral neuropathy, ataxia, parkinsonism |
SPG15 | ZFYVE26 | AR | Defect of autophagy | Complicated HSP with cognitive impairment, peripheral neuropathy, ataxia |
SPG26 | B4GALNT1 | AR | Defect in the biosynthesis of complex gangliosides | Complicated HSP with peripheral neuropathy, mild cognitive impairment, cerebellar and extrapyramidal signs |
SPG28 | DDHD1 | AR | Defect in phospholipid metabolism | Pure HSP |
SPG35 | FA2H | AR | Impaired hydroxylation of sphingolipid fatty acids | Complicated HSP with dystonia, dysarthria, seizures, and mild cognitive decline |
SPG47, SPG50, SPG51, SPG52 | AP4B1, AP4M1, AP4S1, AP4E1 | AR | Deficiency in adaptor protein-4 complex leading to defect of autophagy | Complicated HSP with developmental delay, microcephaly |
SPG49 | TECPR2 | AR | Defect of autophagy | Complicated HSP with developmental delay |
SPG54 | DDHD2 | AR | Defect in phospholipid metabolism | Complicated HSP with cognitive impairment, optic atrophy |
SPG56 | CYP2U1 | AR | Defect in hydroxylation of long-chain fatty acids | Complicated HSP with severe cognitive impairment, dystonia, and peripheral neuropathy |
* AD, autosomal-dominant; AR, autosomal-recessive.
Disorders of Intermediary Metabolism
Disorders in the Metabolism of Vitamins and Cofactors
Homocysteine Remethylation Defects (MTHFR, MMACHC)
This category includes inherited conditions that are caused by defects in the pathway that involves the remethylation of homocysteine to methionine, associated with reduced activity of methionine synthase. These include defects in the synthesis of methylcobalamin, which is a cofactor for methionine synthase, and deficiencies in the supply of the substrate 5-methyltetrahydrofolate. The latter is caused by mutations in the MTHFR gene that encodes methylenetetrahydrofolate reductase (MTHFR), which is involved in the production of 5-methyltetrahydrofolate, while defects in the synthesis of methylcobalamin are part of the more complex genetic disorders of intracellular cobalamin (Cbl) metabolism (Figure 29.1a). One of the most common inherited errors of cobalamin (vitamin B12) metabolism is caused by mutations in the MMACHC gene, which leads to combined methylmalonic acidemia and homocystinuria, cblC type [14, 15].
Figure 29.1 Metabolic pathways involved in the most common HSP-related IEMs. (a) Homocysteine remethylation defects include a deficiency of methylenetetrahydrofolate reductase (MTHFR), which is involved in the production of the substrate 5-methyltetrahydrofolate (5-MTHF). This is necessary for the remethylation of homocysteine to methionine, and complex defects of intracellular cobalamin (CbI) that lead to impaired production of MeCbI, which acts as a cofactor for the remethylation. MTHFR deficiency leads to increased levels of homocysteine, while complex defects, including cbIc deficiency, lead to the combined elevation of homocysteine and methylmalonic acid levels. (b) Urea cycle disorders include arginase deficiency (ARG1) and ornithine translocase 1 (ORNT1) deficiency that causes HHH syndrome. The reaction catalyzed by arginase is the last step in the urea cycle and its deficiency leads to increased levels of arginine and, rarely, to hyperammonemia, while ORNT1 is a mitochondrial transporter and its deficiency causes the typical combination of hyperornithinemia, hyperammonemia, and homocitrullinuria. (c) Dopamine synthesis defects include deficiency of tyrosine hydroxylase (TH), which is the rate-limiting enzyme of catecholamine biosynthesis, or deficiency of the enzymes involved in the biosynthesis of tetrahydrobiopterin (BH4), which is a necessary cofactor for TH, and include GCH1, SPR, and PTPS. (d) Peroxisomal disorders include adrenoleukodystrophy, caused by deficiency of the peroxisomal transporter protein ATP binding cassette subfamily D member 1 (ALDP), leading to the accumulation of very long chain fatty acids (VLCFAs) (e) Lysosomal disorders include metachromatic leukodystrophy caused by a deficiency of arylsulfatase A (ARSA), and Krabbe disease caused by a deficiency of galactosylceramidase (GALC). Both are lysosomal enzymes and they are involved in the metabolism of sulfatides and galactocerebroside. Additional abbreviations: AdoCbI, adenosylcobalamin; ASL, argininosuccinic acid lyase ; ASS, argininosuccinic acid synthetase; DHPR, dihydropteridine reductase; GCH1, GTP cyclohydrolase 1; OHCbI, hydroxycobalamin; OTC, ornithine transcarbamylase; PCD, pterin-4a-carbinolamine dehydratase; PTPS, 6-pyruvoyltetrahydrobiopterin synthase; SPR, sepiapterin reductase; TH, tyrosine hydroxylase; THF, tetrahydrofolate; VLCFA, very long chain fatty acid.
Both conditions are inherited in an autosomal-recessive pattern. More than 100 mutations have been associated with MTHFR deficiency. No genotype–phenotype correlations have been established, although cases with later onset seem to be associated with residual enzymatic activity [16]. More than 50 mutations in MMACHC have been linked with cblC disease and the genotype seems to correlate with the phenotypic classification to early- and late-onset forms [17].
There is incomplete understanding of the underlying pathophysiology, and possible theories include an accumulation of toxic metabolites, oxidative stress, and impaired enzymatic or non-enzymatic protein functions [18]. Although the clinical manifestations of homocysteine remethylation defects can be severe and life-threatening in infantile-onset forms, later-onset cases in childhood, adolescence, or even adulthood have also been reported and are characterized by a complex phenotype, consisting of neurological and hematological manifestations. Neurological symptoms seem to arise due to progressive demyelination affecting the long tracts and causing a clinical presentation that resembles a complex form of HSP [19].
Most cases of MTHFR deficiency present in infancy or childhood. The condition is often characterized by severe encephalopathy, hypotonia, failure to thrive, microcephaly, seizures, and coma, frequently leading to early demise. When the condition manifests after the first year of life the presentation is more insidious with hematological manifestations, usually thrombotic events, and neurological manifestations, including developmental delay and later regression, epilepsy, peripheral neuropathy, and spastic paresis. Cases with onset in adolescence or adulthood have also been identified. Here, the presentation is quite heterogeneous and the phenotype often resembles a complicated slowly progressive HSP, which is commonly preceded or accompanied by cognitive, psychiatric, or behavioral symptoms. Cognitive and psychiatric features may present in episodic fashion as psychotic episodes and relapsing encephalopathy. Epileptic seizures have also been reported [16, 18, 20].
The phenotype of cblC disease is characterized by a wide range of manifestations and age at onset of disease. The earlier onset form of cblC deficiency is associated with intrauterine growth retardation, microcephaly, and dysmorphic features. The infantile-onset form of the disease is the most recognized and is typically associated with hypotonia, failure to thrive, difficulties in feeding, and progressive neurological deterioration. Later-childhood- and adult-onset cases have also been reported and occur after the age of 4 years, and may present in any decade of life. Here, manifestations include cognitive decline, psychiatric symptoms, ataxia, and myelopathy with lower limb weakness and a spastic gait. Additionally, these patients often show hematological complications and thromboembolic events [17, 21].
Brain MRI is usually significant for non-specific white matter abnormalities, usually affecting the posterior areas of the brain. Patients with cblC disease do not have specific radiological findings, but brain atrophy and white matter changes have been reported, particularly in later-onset cases [22].
Homocysteine remethylation defects can be diagnosed by specific changes of laboratory markers. The hallmark feature is hyperhomocysteinemia with levels of >50 μmol/L, and usually >100 μmol/L. In MTHFR deficiency, additional markers include homocystinuria and increased plasma cystathionine with low or normal plasma methionine. Enzymatic activity can also be measured with a direct assay, although this is difficult to perform. Additionally, MTHFR deficiency is characterized by the absence of methylmalonic aciduria, which is commonly present in complex cobalamin metabolism disorders, including cblC deficiency, along with increased plasma methylmalonic acid, homocysteine, and cystathionine. Final confirmation of the diagnosis is made by the identification of pathogenic mutations in the associated genes. Prenatal molecular testing is possible [17, 18].
MTHFR deficiency can be well managed with combined supplementation strategies. This includes the administration of vitamins B12 and B9, in order to increase methionine synthesis, combined with a supplementation of methionine and betaine, which is a substrate for an alternative pathway of homocysteine remethylation to methionine. These therapeutic strategies seem to be effective in children and adults, resulting in an inhibition of the progression, or even sometimes improvement of existing neurological symptoms [18, 19]. Patients with cblC disease respond well to parenteral hydroxycobalamin (OHCbl), showing a significant improvement in biological markers, and an improvement of neurological and hematological manifestations [21].
Biotinidase Deficiency (BTD)
Biotinidase deficiency represents an inherited defect in the metabolism of biotin, resulting in multiple carboxylase deficiencies. It is inherited in an autosomal-recessive manner and the prevalence is 1 in 60,000 births [23]. The condition causes an abnormal endogenous recycling of biotin, which is an important cofactor for four carboxylases involved in gluconeogenesis, the synthesis of fatty acids, and the catabolism of branched-chain amino acids. Defects in these metabolic pathways have been shown to cause delayed myelination, resulting in several neurological sequelae [24, 25]. There are no clear genotype–phenotype correlations, although a later onset of the disease may be related to the genotype of the condition, especially with missense mutations that allow residual enzyme activity [26].
Biotinidase deficiency usually manifests during infancy or early childhood. Presenting symptoms include a neurocutaneous syndrome with eczematous skin rash, seizures, hypotonia, ataxia, optic atrophy, hearing defects, and developmental delay, as well as respiratory problems. Skin manifestations include alopecia, skin rash due to seborrhea, atopic dermatitis and glossitis. If the condition is left untreated it can lead to coma and death. Some delayed-onset cases have also been reported, occurring in children or even in young adults. In these cases the condition presents mainly with an acute or subacute onset of myelopathy, causing spastic paraparesis, or optic neuropathy, sometimes leading to the misdiagnosis of neuromyelitis optica spectrum disorder. Later-onset cases are associated with residual enzymatic activity and symptoms may be triggered by stress or infections [24, 25, 27].
The diagnosis of biotinidase deficiency is usually made with a direct enzyme assay, showing low or undetectable biotinidase activity (<10% in most severe cases and 10–30% in later-onset cases with partial deficiency) [28]. Additional biochemical markers include elevated blood and CSF lactate and pyruvate levels, as well as elevated urinary excretion of 3-hydroxyisovaleric acid, 3-hydroxypropionate, and 3-methylcrotonyl glycine. MRI can be helpful, particularly in the later-onset presentations, showing diffuse white matter abnormalities suggestive of dysmyelination, brain atrophy, and signs of myelopathy [23]. The confirmation of the diagnosis is based on molecular testing.
The condition is successfully managed with biotin and pyridoxine supplementation, which can result in the prevention of disease progression when administrated early. Unfortunately, replacement therapy does not seem to be equally effective in cases that have already developed severe neurological symptoms due to impaired myelination, resulting in irreversible neurological damage.
Disorders of Amino Acid Metabolism
Urea Cycle Disorders (ARG1, SLC25A1S)
The urea cycle disorders (UCDs) are a group of IEMs, caused by defects in genes encoding the enzymes and transporters that are involved in the ammonia detoxification pathways. This group of disorders is characterized by the accumulation of ammonia and other intermediate metabolic by-products, causing dysfunction in several organs and systems, including the central nervous system. Among the UCDs, some conditions have been reportedly associated with neurological presentations that in some cases may resemble a slowly progressive complicated form of HSP. Two of these conditions are arginase 1 deficiency, caused by mutations in the ARG1 gene, and hyperornithinemia–hyperammonemia–homocitrullinuria (HHH) syndrome, caused by mutations in the SLC25A1S or ORNT1 gene that encodes the mitochondrial ornithine translocase 1 (Figure 29.1b). Both conditions follow an autosomal-recessive mode of inheritance and may present with significant phenotypic variability [29].
Hyperargininemia due to arginase 1 deficiency is distinctive among the UCDs for the relatively rare occurrence of hyperammonemia and infrequent hyperammonemic crises. Arginase 1 deficiency is a very rare condition with an estimated prevalence of 1 in 300,000–2,000,000 live births, but it is nevertheless considered one of the most common reversible causes of spastic paraplegia in children. More than 100 mutations in ARG1 have been associated with hyperarginemia, but no clear genotype–phenotype correlation has been established. Patients with nonsense mutations tend to have more severe disease [30]. The disease onset usually happens during infancy and two distinct forms of presentation have been described so far. The first has an acute onset and episodic presentation with nausea, vomiting, and confusion, usually triggered by high protein intake, similar to other UCDs. Most patients, however, present with a slowly progressive neurological phenotype that consists of spastic paraplegia, cognitive decline, and seizures. Additional clinical features, such as ataxia, dystonia, and extrapyramidal signs, may also be present. Onset can be delayed and many cases are diagnosed during childhood, while rare cases with later initial presentation during adolescence or early adulthood have been reported [31–33]. Given that hyperammonemia is not a prominent feature of the disease, the exact pathogenic mechanism is not yet completely understood, although a neurotoxic role of arginine and its metabolites has been suggested by some studies [34, 35].
HHH syndrome has an estimated prevalence of 1 in 35,000 live births and is also characterized by a variable phenotypic presentation that ranges from mild neurological dysfunction to a severe form with encephalopathy, lethargy, hepatic dysfunction, and seizures. In a systematic review of patients diagnosed with HHH syndrome, four different forms, according to age of onset, were recognized: neonatal (22%), infantile (24%), childhood (44%), and adolescence/adulthood onset (9%). In some cases the diagnosis may be significantly delayed [36]. The clinical presentation can also be separated into acute and chronic forms. The acute presentation is characterized by hyperammonemia that is triggered by a large protein intake. Symptoms consist of nausea and vomiting, ataxia, seizures, confusion, lethargy, or even coma. The chronic presentation is characterized by slowly progressive neurological dysfunction that includes pyramidal signs and lower limb spasticity, learning disabilities, cerebellar signs, and seizures. Episodes of confusion and lethargy may also occur. Many of these patients show a chronic aversion to protein intake that seems to act in a protective fashion [36–38].
On laboratory testing, arginase 1 deficiency is characterized by hyperargininemia, while SLC25A1S mutations result in the typical combination of hyperornithinemia, hyperammonemia, and homocitrullinuria. Increased plasma ammonia may be associated with abnormal liver function tests in HHH syndrome, especially during attacks, while they are usually normal in arginase 1 deficiency. Brain MRI may reveal diffuse white matter abnormalities or atrophy in some patients. The diagnosis can be assisted by enzymatic assays and confirmation is made by genetic testing [29].
Both conditions are well managed with a restriction of protein intake and supplementation of essential amino acids. Patients with arginase 1 deficiency who are diagnosed and treated early generally remain asymptomatic. Patients with HHH syndrome are additionally treated with citrulline and arginine supplementation, both in acute crises, as well as for long-term therapy [29].
Phenylketonuria (PAH)
Phenylketonuria (PKU) is an autosomal-recessive inborn error of metabolism, caused by the deficiency of the enzyme hepatic phenylalanine hydroxylase (PAH), which is responsible for converting phenylalanine to tyrosine. The resulting accumulation of phenylalanine and its metabolites affects the development of the central nervous system. The prevalence of PKU is one of the highest among the various IEMs, and its incidence is estimated to be around 1:10,000 in Caucasians, although this varies in different populations [39]. Almost 1,000 different mutations in the PAH gene have been associated with the condition, the majority being missense mutations. A correlation between the genotype, the residual activity of the enzyme, and the clinical presentation has been confirmed by several studies [40].
PKU usually presents during infancy or early childhood and is characterized by a severe phenotype that includes developmental delay, cognitive decline, and seizures. Neurological damage tends to be irreversible if the condition is left untreated and such cases may present in childhood with intellectual disability, epilepsy, and pyramidal and extrapyramidal signs. In rare cases, PKU presents as a later-onset progressive spastic paraparesis with or without cognitive decline, visual disturbances, ataxia, and parkinsonism. In many instances deterioration has been reported to occur in an acute or subacute manner. Cases of reversible but progressive spastic paraparesis in adult PKU patients have been identified, especially after discontinuing or relaxing the low phenylalanine diet. Finally, many treated cases can still show attenuated neurological signs, such as tremor, brisk tendon reflexes, poor motor coordination, or epilepsy [40, 41].
Brain MRI can be characteristic in untreated cases, showing typically diffuse white matter abnormalities, predominantly in posterior periventricular regions, and extending to subcortical and frontal regions in more severe cases. The diagnosis is established by the demonstration of elevated serum phenylalanine levels. Additional diagnostic markers include low serum tyrosine levels, and the presence of phenylacetate in the urine. Systematic newborn screening for PKU, established since the second half of the twentieth century in many countries, has significantly reduced the occurrence of undiagnosed and untreated cases, although false negatives in the initial screening may still occur [39].
A low phenylalanine diet is the standard treatment for PKU, while an alternative treatment is the administration of tetrahydrobiopterin (BH4), which is a required cofactor for PAH. Additional potential therapeutic strategies include using benzylhydantoin instead of BH4 and a drug called phenylalanine ammonia lyase (PAL) that is useful in reducing the levels of phenylalanine in the plasma. Many of the patients who present with a later onset of the disease respond well to treatment and usually show significant improvement of the neurological symptoms [42].
Dopamine Synthesis Defects (GCH1, TH, SPR, PTPS)
Mutations in genes related to dopamine synthesis are typically associated with dopa-responsive dystonia (DRD). This group of disorders includes defects in the biosynthesis of BH4 or in rate-limiting enzymes of catecholamine biosynthesis: (i) GTP cyclohydrolase 1 (GTPCH1) deficiency, (ii) tyrosine hydroxylase (TH) deficiency, (iii) sepiapterin reductase (SPR) deficiency, and (iv) 6-pyruvoyltetrahydrobiopterin synthase (PTPS) deficiency (Figure 29.1c). Autosomal-dominant GCH1 mutations were first identified in cases with DRD, also known as Segawa disease or DYT5a dystonia. Subsequently, autosomal-recessive mutations in GCH1, as well as in TH, SPR, and PTPS genes have been associated with a similar phenotype, as well as with a wider and more complex range of neurological symptoms. In the latter cases, spasticity is a prominent clinical feature. The term DRD-plus has been proposed to describe these atypical presentations [43–45].
Classic DRD is characterized by childhood or adolescent onset of dystonia with diurnal fluctuations and mild parkinsonism, that responds well to levodopa. Dystonia initially often affects the lower limbs, presenting with toe walking and frequent falls, while brisk tendon reflexes are not uncommon. Atypical DRD, or DRD-plus syndromes, include cases of juvenile parkinsonism, ataxia, and spastic paraplegia [46]. Later-adulthood-onset cases have also been reported, presenting with parkinsonism, focal dystonia, or, occasionally, with no dystonia at all [45].
The most common cause of DRD is autosomal-dominant GCH1 mutations and patients usually show an excellent and sustained response to levodopa. Autosomal-recessive GCH1 patients may present with more complex phenotypes, usually in infancy, that include limb spasticity, axial hypotonia, oculogyric crises, and delay in motor and cognitive development. Some of these patients show only a partial response to levodopa. TH deficiency has a wide clinical spectrum ranging from severe progressive encephalopathy to typical DRD. Complex DRD-plus syndromes have also been reported with a few cases resembling spastic paraplegia. SPR deficiency may present as typical DRD or a more complex DRD-plus with spasticity, axial hypotonia, ataxia, oculogyric crises, developmental delay, and cognitive decline. Finally, patients with PTPS deficiency usually have earlier-onset and severe and complex presentations with axial hypotonia, limb spasticity, seizures, psychiatric symptoms, and cognitive defects. These patients may have poor motor outcomes [45, 47–49].
The diagnosis of dopamine synthesis defects is supported by specific changes in the blood and CSF levels of neopterin, biopterin, homovanillic acid, and 5-hyroxyindolacetic acid. Hyperphenylalaninemia may be found in cases with autosomal-recessive GCH1, TH and PTPS mutations. A helpful diagnostic tool in selected cases is the oral phenylalanine loading test, with an increase in the phenylalanine:tyrosine ratios and biopterin concentrations in plasma being diagnostic. Brain MRI usually shows no significant abnormalities.
Treatment is based on the administration of levodopa or dopamine agonists with a good response and long-term benefit in most cases. The response to levodopa varies though and seems to correlate with the associated genotype. Patients that harbor autosomal-dominant GCH1 and SPR mutations overall have the best motor outcomes. A dopamine trial should be offered in all patients with symptoms suggestive of dopamine synthesis defects, even if the presentation might seem atypical [50].
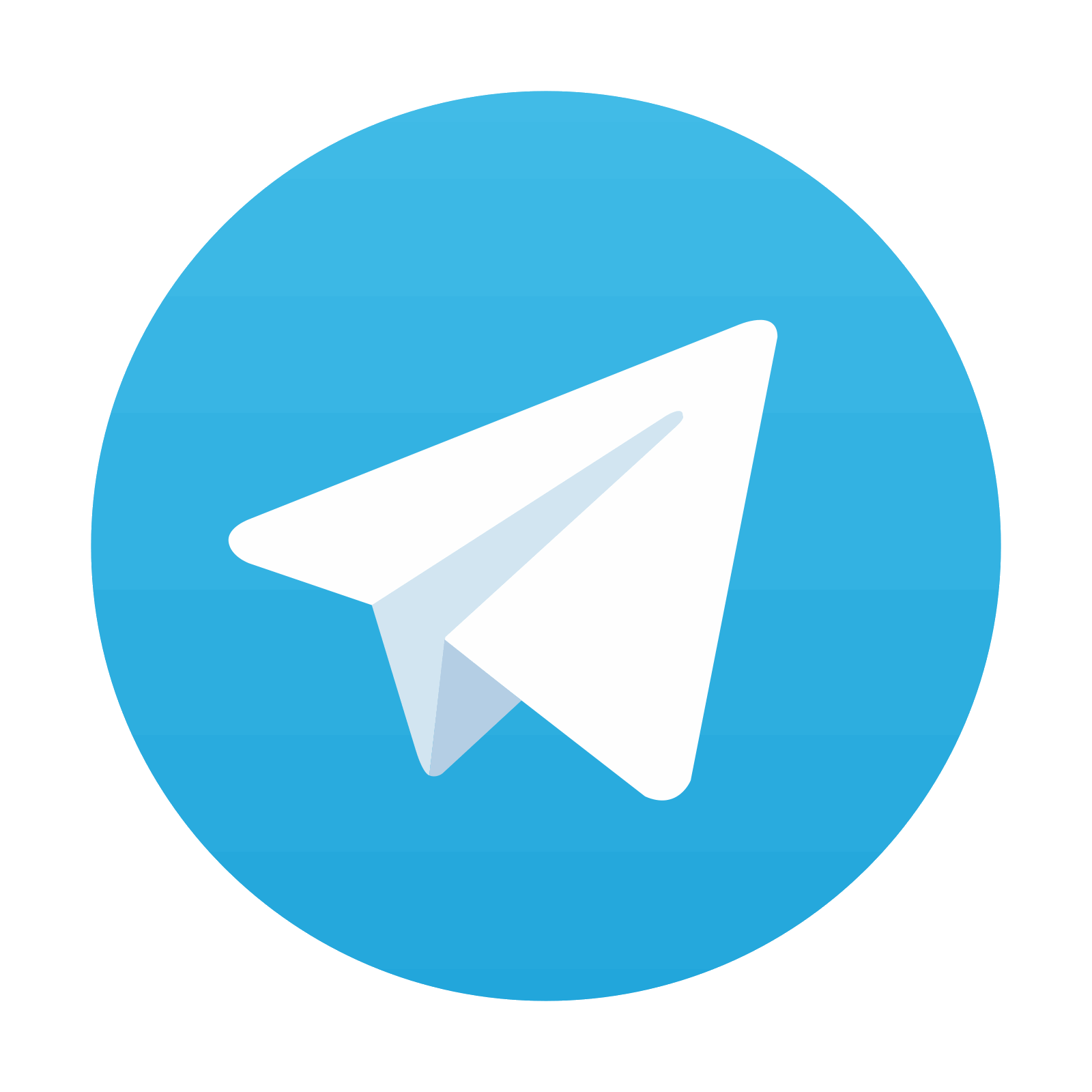
Stay updated, free articles. Join our Telegram channel
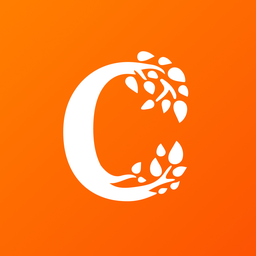
Full access? Get Clinical Tree
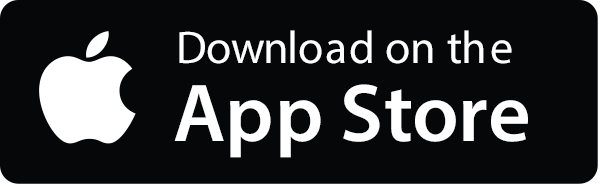
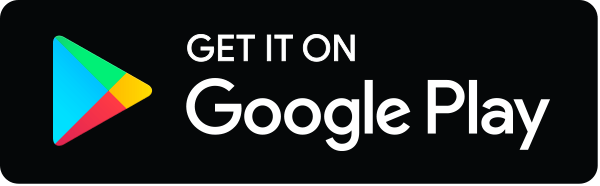
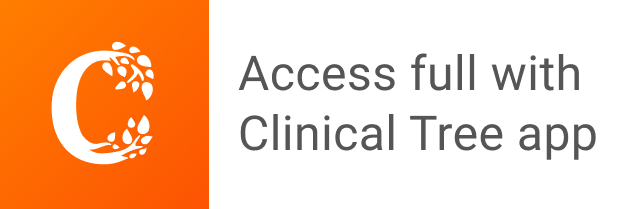