Abstract
During the evaluation of a patient with a movement disorder, advanced neuroimaging is often obtained to narrow the diagnostic possibilities and also to assess the status of what is frequently a neurodegenerative process. In the other chapters of this monograph, specific metabolic causes of movement disorders are discussed in detail. In this chapter, we provide a pragmatic approach for integrating imaging data into the work-up of a patient with a movement disorder of uncertain etiology, heavily emphasizing brain MRI which is the cornerstone of imaging work-up for this indication. Our focus is on pediatric-onset disorders manifesting as dystonia, chorea, athetosis, tremor, parkinsonism, and myoclonus.
Introduction
During the evaluation of a patient with a movement disorder, advanced neuroimaging is often obtained to narrow the diagnostic possibilities and also to assess the status of what is frequently a neurodegenerative process. In the other chapters of this monograph, specific metabolic causes of movement disorders are discussed in detail. In this chapter, we provide a pragmatic approach for integrating imaging data into the work-up of a patient with a movement disorder of uncertain etiology, heavily emphasizing brain MRI which is the cornerstone of imaging work-up for this indication. Our focus is on pediatric-onset disorders manifesting as dystonia, chorea, athetosis, tremor, parkinsonism, and myoclonus. Since spasticity is a common end-state of many neurodegenerative conditions, it is not emphasized in this review, and likewise we omit disorders for which the primary manifestation is ataxia. Several review articles provide complementary information on adult-onset metabolic movement disorders or imaging algorithms for metabolic conditions or leukodystrophies more generally [1–6].
We begin by reviewing a standard brain MRI protocol for a patient undergoing evaluation of a movement disorder, and we briefly detail the normal developmental trajectory of the brain, since many metabolic conditions present at a time of ongoing developmental changes in brain signal (e.g. myelination). Metabolic conditions which cause movement disorders are then discussed according to imaging patterns: basal ganglia T2 hyperintensity, abnormal basal ganglia mineralization, diffuse white matter signal abnormality, and disorders without specific brain imaging findings. We contrast these disorders with acquired conditions which may mimic metabolic movement disorders, either on imaging or by clinical presentation. A graphical overview of the approach in this chapter is provided in Figure 4.1.
Figure 4.1 Proposed diagnostic approach to movement disorders based on imaging findings: (1) basal ganglia T2 hyperintensity; (2) mineralization of the basal ganglia; (3) diffusely abnormal white matter signal; (4) normal or non-specific atrophy.
Imaging for Movement Disorders and Normal Brain Development
A brain MRI obtained for evaluation of a movement disorder will usually be protocoled to screen broadly for underlying injuries, neoplasm, and evidence of a genetic condition. While a number of protocols can be devised to evaluate these possibilities, our institutional protocol for evaluation of a patient with movement disorders (Box 4.1) includes isotropic T1-weighted imaging, high resolution axial/coronal T2-weighted imaging, susceptibility-weighted imaging (SWI), and diffusion tensor imaging. For patients older than 6–12 months, axial fluid-attenuated inversion recovery (FLAIR) imaging will also be routinely performed, and since studies are monitored in real time in our practice, there is always the option of injecting contrast should the non-contrast imaging warrant it. If there is a strong suspicion of a metabolic condition as the basis of a movement disorder, multivoxel MRS at short and intermediate echo times is also obtained, and in cases where deep brain stimulator placement is likely to be recommended, surgical navigation sequences as well as an MR angiogram will be obtained. The described imaging protocol is of sufficient detail to detect congenital structural abnormalities (e.g. polymicrogyria), injuries (e.g. hypoxic ischemic insult), neoplasms, and patterns of signal abnormality typical of particular genetic conditions (discussed below).
3 Tesla MRI
32- or 64-channel head coil
Sagittal T1 MPRAGE (magnetization-prepared rapid gradient echo) or IR-SPGR (fast spoiled GRASS sequence with IR preparation) at isotropic resolution (0.9 mm)
Axial T2 (0.4 × 0.5 × 2.5 mm skip 0 mm)
Coronal T2 (0.4 × 0.5 × 2.5 mm skip 0 mm)
Axial FLAIR (0.6 × 0.6 × 4 mm skip 1 mm)
Axial susceptibility weighted imaging (SWI), susceptibility weighted angiography (SWAN), or venous blood oxygen level dependent (VEN-BOLD) (0.8 × 0.9 × 1.25 mm)
Three-dimensional spiral chemical shift imaging MR spectroscopy (30 ms, 135 ms echo time)
Simultaneous multislice diffusion tensor imaging (35 directions, 1.5 × 1.5 × 2 mm skip 0 mm)
T1, T2, and SWI characteristics of the brain evolve throughout childhood due to normal myelination and physiological iron deposition. A basic understanding of these changes is therefore essential to detecting abnormalities on brain MRI in young patients.
Dramatic changes in brain myelination occur during the first 2 years of life, most conspicuous on T1-weighted sequences during the first 7–8 months and later most conspicuous on T2-weighted imaging. With myelination, any given brain structure develops increased T1 hyperintensity and decreased T2 hyperintensity, which can be conceptualized as a transition from watery to more proteinaceous brain matter. Complex tables listing normative myelination milestones for specific brain structures are available [7], but an understanding of brain myelination at three early time points usually suffices for most day to day evaluations of brain myelination (Figure 4.2a–f). At birth (Figure 4.2a, b), the brain is largely unmyelinated apart from small areas of myelinated brain (high T1 and low T2 signal) in the ventrolateral thalamus, posterior putamen, and posterior limb of the internal capsule; the dorsal brainstem, perirolandic cortex, and primary visual cortex are also myelinated at birth. By 6–8 months of age, myelination on T1-weighted imaging has reached the subcortical white matter (Figure 4.2c) though myelination on T2-weighted imaging is limited to the internal capsule and corpus callosum (Figure 4.2d); myelination of the posterior fossa structures will be complete on both T1 and T2, having typically reached maturity by 3 months. By 18–20 months, the myelination in the subcortical white matter has further matured on T1 imaging (Figure 4.2e) and the myelination on T2 imaging has reached the subcortical white matter (Figure 4.2f). Thereafter, minimal incremental myelination will occur in the subcortical white matter (perceptible changes through the first 5 years) and the periatrial white matter (periatrial “terminal zones” can persist through adolescence). An important consequence of this evolution of signal intensity is that T2 hyperintensity can be obscured during the first 2 years of life due to the intrinsically T2 hyperintense signal of unmyelinated brain parenchyma, and conversely pathological T2 hyperintensity of the globus pallidus can be falsely suggested around 6–8 months due to relative differences in the rates of internal capsule versus basal ganglia myelination (Figure 4.2d). As illustrated in specific cases discussed later, these limitations can be partly addressed using diffusion imaging.
Figure 4.2 Normal brain myelination using T1-weighted (a, c, e) and T2-weighted (b, d, f) sequences. In the normal neonatal brain (a, b), there is T1 hyperintensity and T2 hypointensity from brain myelination at the ventrolateral thalamus (arrow with solid stem on the left), posterior putamen (arrow with dashed stem on the left), and posterior limb of the internal capsule (asterisk on the left). By 6 months of age (c, d), myelination is now visible out to the subcortical white matter throughout the brain on T1-weighted imaging but myelination on T2-weighted imaging is limited to the internal capsule and corpus callosum. Note relative T2 hyperintensity of the globus pallidus at this age (asterisk on the left in d). By 18 months of age (e, f), myelination has further matured in the subcortical white matter on T1-weighted imaging, and myelination on T2-weighted imaging has reached the subcortical white matter.
With SWI, local disturbances in tissue magnetization (e.g. from iron or calcium deposition) result in a loss of MRI signal. As typically implemented, SWI sequences employ a gradient echo sequence that has both T1 and T2 weighting [8, 9], providing a relatively low-contrast image of the normal brain with the exception of brain nuclei with physiological mineralization: globus pallidi, subthalamic nuclei, substantia nigra, red nuclei, and dentate nuclei. In these locations, there is no detectable mineralization at birth but faint physiological iron deposition is seen by school age and this increases with age (Figure 4.3). Normative measures of this process are scarce [10–12]. Therefore, assessing for abnormal iron deposition in brain nuclei is a somewhat subjective exercise on routine clinical imaging, reflecting scanner-specific effects as well as interindividual variation. Calcification also accumulates in the basal ganglia with age and can be detected on SWI. As with iron deposition, normative data are scarce though it is rare to see calcification in the basal ganglia before adolescence [13, 14]. By clinical experience, calcifications are more likely to manifest as focal deposits than diffuse deposition throughout a deep brain nucleus, for example as seen in neurodegeneration with brain iron accumulation (NBIA). Nonetheless, there are instances where confusion about calcium versus iron deposition may persist on MRI. In those cases, the increased magnetization associated with iron and decreased magnetization associated with calcium deposits can be separated using detailed “phase” maps from SWI (Figure 4.4) [15]. CAT scan is a complementary means of detecting calcification.
Figure 4.3 Normal brain mineralization and characterization of calcium versus iron using SWI. At 5 years (a, b), 10 years (c, d), and 19 years (e, f) of age, there is progressive physiological iron deposition seen at the level of the basal ganglia (a, c, e) and midbrain (b, d, f). Left-sided nuclei are labelled in e, f: GPi, globus pallidus internus; GPe, globus pallidus externus; r, red nuclei; and SN, substantia nigra.
Figure 4.4 Characterization of mineralization by SWI. Susceptibility in a right frontal lesion (a) is characterized by the SWI phase map (b) as opposite phase to blood dephasing (i.e. black rather than white). The phase map enables inference of calcification as the basis of the susceptibility, suggesting that the small mass seen on T2-weighted imaging (c) is calcified as confirmed by non-contrast head CT (d). Conversely, the SWI phase map for a patient with a subacute basal ganglia infarct (e) demonstrates that susceptibility (f) in the infarct seen on T2-weighted imaging (g) represents petechial blood (white on phase map).
The relative concentration of metabolites detectable on MRS also changes with age, slowly reaching an adult pattern by 2 years of age [7]. In practice, this changing relationship has a limited application to metabolic movement disorders where the presence of abnormal metabolites (e.g. lactate) or the absence of normal metabolites (e.g. creatine) is generally more important than quantitative relationships.
Neuroimaging Patterns in Metabolic Movement Disorders
The neuroimaging patterns in inborn errors of metabolism (IEMs) associated with movement disorders can be divided into four general categories: (1) symmetrical T2 hyperintensity of basal ganglia; (2) mineralization of basal ganglia; (3) diffuse abnormalities of cerebral white matter signal; and (4) normal or non-specific patterns.
Symmetrical T2 Hyperintensity of Basal Ganglia
Symmetrical T2 hyperintensity of the basal ganglia is a common neuroimaging pattern in a number of IEMs associated with movement disorders, including organic acidemias, pyruvate dehydrogenase (PDH) deficiency, mitochondrial disorders more generally, infantile forms of GM1 and GM2 gangliosidoses, Wilson’s disease, and guanidinoacetate N-methyltransferase (GAMT) deficiency.
Organic Acidemias
The organic acidemias are a diverse group of genetic disorders caused by enzymatic deficiencies in the catabolic pathways of amino acids, resulting in the accumulation of specific organic acids in body fluids. They can be divided clinically into classic organic acidemias that present with metabolic derangements in blood, including ketoacidosis and hyperammonemia, in addition to neurological symptoms, and cerebral organic acidemias that typically manifest with only neurological symptoms. Examples of classic organic acidemias include propionic acidemia, methylmalonic acidemia, isovaleric acidemia, and beta-ketothiolase deficiency. Examples of cerebral organic acidemias include glutaric acidemia type 1, 3-methyl-3-hydroxybutyric acidemia, L2-hydroxyglutaric acidemia, 3-methylglutaric acidemia, and succinate semialdehyde dehydrogenase deficiency. A specific diagnosis is made by measuring urine organic acids, and in some disorders the plasma acylcarnitine profile [16].
The clinical presentations of the various organic acid disorders are distinct. Propionic and methylmalonic acidemia present with recurrent ketoacidosis and hyperammonemia, provoked by high protein load or metabolic stressors like prolonged fasting or illness. Developmental delay, failure to thrive/anorexia, and gastrointestinal dysmotility are common complications. Pancreatitis, sensorineural hearing loss, and optic neuropathy have also been reported. Chronically, patients with propionic acidemia may develop cardiomyopathy and QT prolongation, whereas patients with methylmalonic acidemia may develop renal failure. Patients with glutaric acidemia type 1 are often macrocephalic since infancy. They are typically asymptomatic initially, but are at risk for metabolic stroke in the first 6 years of life, provoked by increased metabolic stress, such as febrile illness. They are also susceptible to subdural hemorrhages due to enlarged extra-axial spaces, which can mimic non-accidental trauma. The most common movement disorder seen in the organic acidemias is dystonia, but choreoathetosis, parkinsonism, and myoclonus have also been reported. These movement abnormalities may develop subacutely following a metabolic stressor such as illness or infection, or more insidiously [16].
In the organic acidemias as a group, neuroimaging may be normal prior to an acute central nervous system event or abnormalities may develop insidiously. The most common pattern of signal abnormality is T2 hyperintensity of the basal ganglia that is almost always symmetric, although unilateral or asymmetrical cases have been encountered. The specific pattern of basal ganglia involvement and whether or not there is additional involvement of substantia nigra pars reticulata, cerebellar dentate nuclei, and cerebral white matter is disease specific (summarized in Table 4.1) [17–21]. There may also be a diffusion restriction of affected structures in the acute stages [22]. Examples of brain MRI of patients with organic acidemias are presented in Figures 4.5–4.7. Spectroscopy is normal or may demonstrate lactate elevation.
Table 4.1 Neuroimaging findings in the organic acidemias
Basal ganglia/ substantia nigra | White matter | Other | |
---|---|---|---|
Propionic acidemia | Striatum, substantia nigra | Subcortical U-fibers | No |
Methylmalonic acidemia | Globus pallidi, substantia nigra reported | Non-specific gliosis | Dorsal brainstem, cerebellar hemispheres, volume loss |
Isovaleric acidemia | Globus pallidi | Yes, non-specific | No |
Beta-ketothiolase deficiency | Variable | No | |
Glutaric acidemias type 1 | Striatum | Yes, non-specific | Central tegmental tracts, dentate, thalami, macrocephaly, open operculum, subdural fluid collections |
L2-hydroxyglutaric acidemias | Globus pallidus | Subcortical U-fibers (predominantly frontal) | Dentate |
Figure 4.6 12-year-old male with methylmalonic acidemia, global developmental delay, intractable seizures, and new tremors. (a) Axial FLAIR images demonstrate signal abnormality in the basal ganglia and patchy periventricular gliosis, new findings compared to a study performed 4 years prior. (b) Axial apparent diffusion coefficient maps indicate that the signal abnormality has increased diffusivity in keeping with a subacute to chronic metabolic insult.
Mitochondrial Disorders
Mitochondrial disorders encompass a wide range of genetic defects that affect the assembly of the mitochondrial respiratory chain, function of the citric acid cycle, mitochondrial translation, mitochondrial maintenance, mitochondrial fission/fusion, intra-mitochondrial transport, mitochondrial cofactor synthesis/metabolism, and autophagy. Mitochondrial disorders can result from defects in nuclear or mitochondrially encoded genes. The phenotypic spectrum of mitochondrial disorders is highly pleiomorphic, and may involve vision, hearing, the heart, liver, kidney, endocrine system, central nervous system, and peripheral nervous system. A wide range of movement disorders have been described in mitochondrial disorders, including dystonia, choreoathetosis, parkinsonism, tremor, ataxia, and myoclonus. Biochemical evaluation may demonstrate elevations in lactic acid with increased lactate:pyruvate ratio, elevations in plasma alanine and/or proline, abnormalities of citric acid cycle metabolites, or the accumulation of specific organic acids in urine. Some mitochondrial disorders have more specific biochemical abnormalities (e.g. elevated lactate with a normal (<20) lactate:pyruvate ratio in PDH deficiency and low plasma arginine in MELAS [mitochondrial encephalomyopathy with lactic acidosis and stroke-like episodes] syndrome) [16].
Imaging in mitochondrial disorders is variable, but a common pattern involves symmetrical T2 hyperintensity of deep gray nuclei and brainstem tracts [23–25]. This pattern was initially described, based on specific post-mortem pathology findings, as Leigh syndrome; however, when this distribution of signal abnormality is seen in vivo on MRI, it is best described as “Leigh-like.” In addition to an increased T2 signal, the involved structures may show cavitation and necrosis. Affected structures may also demonstrate diffusion restriction, but unlike the typical diffusion changes following ischemia, they may be prolonged (lasting months) in mitochondrial disorders, and may not result in gliosis or atrophy [23]. MRS may demonstrate a lactate doublet at 1.3 ppm that is upright at short and long echo times, and inverts at intermediate echo times. Examples of mitochondrial disorders are shown in Figures 4.8 and 4.9.
Figure 4.8 PDH deficiency in a 28-month-old child with developmental delay and seizures. (a) Axial T2 and (b) diffusion-weighted images demonstrate diffusion restriction and T2 hyperintensity within the globus pallidi.
Figure 4.9 A 1-year-old male with POLG-associated Alpers syndrome, manifest as refractory status epilepticus, myoclonus, and liver failure. (a) Axial T2-weighted imaging demonstrates thalamic T2 hyperintensity and posterior quadrant cortical/subcortical T2 prolongation, much more conspicuous on (b) an axial diffusion trace and (c) apparent diffusion coefficient (ADC) maps.
Additional neuroimaging patterns in mitochondrial disease include stroke-like, cortically based lesions that cross vascular territories (e.g. mitochondrial encephalomyopathy with lactic acidosis and stroke-like episodes [MELAS] syndrome, Alpers syndrome); non-specific cerebral and/or cerebellar atrophy; and prominent white matter signal alterations (e.g. Kearn–Sayre syndrome, mitochondrial neurogastrointestinal encephalopathy syndrome, disorders of mitochondrial amino-acyl transfer RNA synthetases, some complex 1 defects). Mitochondrial disorders may occasionally be associated with calcification of the basal ganglia, and brain malformations (e.g. callosal dysgenesis in pyruvate dehydrogenase deficiency, migrational abnormalities in fumarase deficiency) [5, 24].
One disorder that deserves special attention in this category since it is amenable to therapy is biotin–thiamine-responsive basal ganglia disease [26]. Patients present with episodes of subacute encephalopathy, seizures, ataxia, dystonia, rigidity, and brainstem signs provoked by metabolic stressors. Occasionally, the presentation can be chronically progressive. Neuroimaging demonstrates bilateral T2 hyperintensity in caudate heads, putamen, and, occasionally, globi pallidi, thalami, brainstem tracts, cerebellum, cortex, and subcortical white matter (Figure 4.10). Patients respond favorably to early administration of high-dose biotin and thiamine.
Figure 4.10 Biotin–thiamine responsive basal ganglia disease at 12 months. (a) Axial T2 and (b) FLAIR images demonstrate bilateral striatal necrosis. The patient had a history of paroxysmal episodes of weakness and ataxia responsive to a mitochondrial cocktail including biotin.
Infantile (Acute) Forms of GM1 and GM2 Gangliosidoses
GM1 and GM2 gangliosidoses are caused by defects in lysosomal hydrolases that result in an accumulation of ganglioside substrates (a form of sphingolipid) within lysosomes [6]. GM1 gangliosidosis results from a deficiency of the enzyme beta-galactosidase; GM2 gangliosidosis type 1 (Tay–Sachs disease) results from a deficiency of the enzyme hexosaminidase A, GM activator protein, or saposin A; and GM2 gangliosidosis type 2 (Sandhoff disease) results from a deficiency of the enzyme hexosaminidase B. The gangliosidoses can be further divided into several subtypes based on the age of onset and rate of disease progression, including infantile/acute, juvenile/subacute, and adult/chronic forms. A specific diagnosis can be made through enzyme analysis in blood or fibroblasts [16].
The infantile forms of both GM1 and GM2 gangliosidoses present in the first year of life with rapidly progressive neurodegeneration, resulting in encephalopathy and spastic quadraplegia. There may be a macular cherry red spot from lipid storage in perimacular retinal cells. In addition, in GM1 gangliosidosis and the infantile form of GM2 gangliosidosis type 2 (Sandhoff disease), there may also be extra-neurological features, including hepatosplenomegaly, skeletal anomalies, and coarsening of facial features. Movement disorders include startle-provoked myoclonus and, in the terminal stages, decerebrate posturing [16].
Neuroimaging in infantile GM1 and GM2 gangliosidoses is notable for delayed myelination which may be accompanied by basal ganglia T2 hyperintensity. The thalami demonstrate hyperdensity on CT and relative T2 hypointensity on MRI (Figure 4.11) [27, 28].
Figure 4.11 GM1 gangliosidosis in a 9-month-old infant with motor delay, hypotonia, hepatomegaly, rickets-like changes on radiographs, and cherry red spots on ophthalmological examination. (a) Axial T1 and (b) axial T2 images demonstrate a markedly delayed myelination pattern for a patient of this age. (c) There is also a question of relative T2 hypointensity of the thalami, correlating with hyperdensity of the thalami on CT, 3 months later.
Wilson’s Disease
Wilson’s disease results from defective incorporation of copper into ceruloplasmin and inadequate excretion of biliary copper, producing systemic copper overload. Neurological features include progressive parkinsonism, dystonia (particularly bulbar), “wing-beating” tremor, dementia, and psychiatric symptoms. Extra-neurological features include liver disease, hemolytic anemia, Kaiser–Fleischer rings from accumulation of copper in Descemet’s membrane of the eye, and, rarely, cardiomyopathy and renal tubulopathy. Onset may be from childhood to adulthood; childhood forms tend to be dominated by hepatic involvement. Serum copper and ceruloplasmin are often reduced; 24-hour urine copper is increased; and liver biopsy may demonstrate hepatic copper accumulation [16].
While imaging may be normal in presymptomatic patients, typical imaging findings in symptomatic patients include basal ganglia T2 hyperintensity, cerebral atrophy, and brainstem/thalamic signal abnormalities [29]. The brainstem signal abnormality tends to concentrate in the midbrain with near universal involvement of the tectum and less frequent involvement of the midbrain tegmentum (when associated with sparing of the red nuclei, the midbrain tegmentum signal abnormality is dubbed the “giant panda sign”) [30]. Co-occurrence of the basal ganglia susceptibility artifact or T1 hyperintensity can sometimes be seen in Wilson’s disease, possibly as a result of parenchymal calcification or manganese deposition in the setting of liver dysfunction, respectively [31]. A typical case of adolescent onset Wilson’s disease is depicted in Figure 4.12.
Figure 4.12 Wilson’s disease in a 12-year-old adolescent with tremor, micrographia, and dysarthria. Axial T2-weighted images demonstrate T2 hyperintensity within (a) the corpus striatum and (b) the dorsal brainstem.
Guanidinoacetate N-Methyltransferase Deficiency
GAMT is the second enzyme in the biosynthetic pathway of creatine. GAMT deficiency is associated with developmental delay, particularly affecting language, epilepsy, behavioral abnormalities, and a complex movement disorder that may include chorea, athetosis, dystonia, and ataxia. MR may demonstrate T2 hyperintensity of bilateral globi pallidi [32]. Spectroscopy demonstrates a reduced creatine peak. Of note, the other known disorders of creatine metabolism, namely arginine glycine amidinotranserase (AGAT) deficiency and the creatine transporter defect, do not generally show abnormalities on structural MRI, but do show reduced creatine on MRS (Figure 4.13).
Figure 4.13 A 4-year-old male with developmental delay, hypotonia, and X-linked creatine deficiency secondary to SLC6A8. (a) Axial T2 image at the level of the basal ganglia demonstrates no discrete signal abnormality. (b) Intermediate echo time MRS of the left basal ganglia demonstrates the absence of the normal creatine peak (arrow).
Mimics
While several of the disorders described above have specific imaging findings, it should be emphasized that many non-metabolic genetic and acquired disorders can have similar imaging findings. These mimics can usually be excluded by clinical history and/or biochemical evaluation.
Metabolic/Toxic (Non-Genetic)
Kernicterus in the chronic phase can present with atrophy and T2 hyperintensity in the globus pallidi, subthalamic nuclei, dentate nuclei, and hippocampi, which could be mistaken for an IEM-absent clinical context (Figure 4.14) [33]. Likewise, profound nutritional deprivation can result in Wernicke encephalopathy, typically with signal abnormality along the margins of the third ventricle (thalami, periaqueductal gray matter) as well as the mammillary bodies (Figure 4.15) [34]. Non-ketotic hyperglycemia occurs in individuals with uncontrolled diabetes and presents with movement disorders; on imaging, affected patients typically have unilateral or asymmetrical basal ganglia hyperdensity on CT or T2 hyperintensity on MRI [35]. Drug toxicity can also involve the deep gray matter structures (e.g. methadone or vigabatrin) [36] (Figure 4.16).
Figure 4.14 Kernicterus evaluated at 16 months for generalized dystonia, global developmental delay, hearing loss, and cortical visual impairment. (a) An axial T2 image at the level of the basal ganglia demonstrates abnormal T2 hyperintensity of the globus pallidi. (b) Coronal T2 imaging additionally demonstrates T2 hyperintensity of the subthalamic nuclei (“stn” on the left) and hippocampi (arrow on the left), the latter with volume loss.
Figure 4.15 Wernicke syndrome in an 11-year-old cancer patient receiving chemotherapy and presenting with diffuse weakness and short-term memory loss. Diffusion-weighted imaging demonstrates abnormal signals in the medial thalami and left basal ganglia (a) as well as the mammillary bodies (arrows, b). Involvement of the thalami, periaqueductal gray matter, and mammillary bodies is typical for Wernicke syndrome.
Figure 4.16 A 9-month-old infant with tuberous sclerosis and infantile spasms treated with vigabatrin. Axial diffusion-weighted images demonstrate abnormal signals in: (a) the globus pallidus and thalami; (b) the innominate substance and genu of the internal capsule; and (c) the cerebral peduncles.
Ischemic
Dystonic cerebral palsy due to in utero or perinatal insult can be indistinguishable from end-stage metabolic disease with profound volume loss that encompasses the deep gray matter (Figure 4.17) [37, 38]. Likewise, hypoxic ischemic injury later in life can present with deep gray matter signal abnormality and other abnormalities that depend on the severity of the insult (Figure 4.18).
Figure 4.17 19-year-old patient, former premature infant, with athetosis, dystonia, and spastic quadraparesis. The axial FLAIR image demonstrates marked thinning of the posterior periventricular white matter, minimal periventricular gliosis, and the small size of the thalami. These are typical findings of white matter of prematurity.
Figure 4.18 Hypoxic ischemic injury in an 11-month-old infant with carbon monoxide poisoning and cardiac arrest. (a) Axial T2 image from the admission MRI demonstrates extensive deep gray matter and diffuse hemispheric signal T2 prolongation. (b) At a six-month follow up, there is evolution to extensive deep and cortical gray matter encephalomalacia.
Genetic (Not Metabolic)
Juvenile Huntington disease is unique for frequent T2 hyperintensity in the corpus striatum in addition to the caudate-predominant atrophy seen in older patients (Figure 4.19) [39]. Bilateral infantile striatal necrosis caused by defects in the NUP62 gene can have a similar appearance to mitochondrial disorders but are distinguishable by lack of lactate [40].
Figure 4.19 Juvenile Huntington disease in a 10-year-old child with dysarthria, chorea, and mild ataxia. (a) Axial T2 and (b) coronal T2 images demonstrate marked volume loss and T2 hyperintensity in the corpus striatum (caudate and putamen).
Infectious/Inflammatory
Finally, inflammatory and infectious conditions can cause deep gray matter signal abnormality (e.g. viral encephalitis, acute disseminated encephalomyelitis, acute necrotizing encephalopathy) [41] (Figure 4.20).
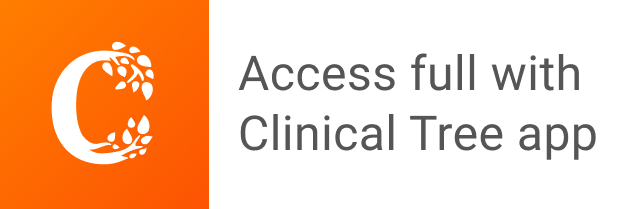