Fig. 1
Schematic of a mouse implanted with optical fibers connected with blue and yellow lasers for combinatorial optogenetic interrogations (Carter et al. 2012)
Optogenetics provided the ideal tools to interrogate causal relationships between the activity of genetically identified neurons and brain states. Before optogenetics, it was difficult to selectively stimulate or inhibit specific hcrt and LC-NE populations with a temporal resolution relevant to sleep or wakefulness episodes and to achieve spatial selectivity to probe those cells without affecting surrounding cells or fibers of passage. This was particularly challenging in species where sleep and wakefulness are not consolidated such as laboratory rodents. Mice and rats have sleep cycles that last between 6 and 12 min and show frequent awakenings during the light (rest) cycle. This temporal scheme makes it impossible to use pharmacological interventions, as these span timescales that extend many sleep/wake cycles .
To deliver the optogenetic probes to hcrt or LC neurons, we used lentiviral and cre-dependent adeno-associated viral (AAV) gene delivery tools, under the control of cell-type specific (Adamantidis et al. 2007). To deliver light to the hcrt or LC field, we designed optical–neural interfaces in which optical fibers were chronically implanted on the mouse skull, as described elsewhere. Using this strategy, we were able to control hcrt neural activity both in vitro and in vivo with millisecond-precise optical stimulation (Adamantidis et al. 2007). The high temporal and spatial precision of stimulation allowed us to mimic the physiological range of hypocretin neuron discharge rates (1–30 Hz) (Hassani et al. 2009). Indeed, we used light-pulse trains for our optogenetic stimulation that were based on parameters on the actual frequency analysis of hcrt neurons in vivo (8–12 Hz). We found that direct unilateral optical stimulation of hcrt neurons increased the probability of transitions to wakefulness from either SWS or REM sleep. Interestingly, high-frequency optical stimulation (5–30 Hz light-pulse trains) reduced the latency to wakefulness, whereas 1 Hz trains did not, suggesting a frequency-dependent synaptic release of neurotransmitter (glutamate) and neuromodulators, including hcrt or dynorphin from the terminals. We further showed that the effects of stimulating hcrt neurons could be blocked by injection of an HcrtR antagonist or by genetic deletion of the hcrt gene, suggesting that hcrt peptides mediate, at least in part, optogenetically induced sleep-to-wake transitions and that fast transmission through glutamatergic terminals of Hcrt cells appears to be dispensable for Hcrt function. These experiments show that hcrt release from hcrt-expressing neurons is necessary for the wake-promoting properties of these neurons. It should be noted, however, that optogenetic stimulations may not reflect the actual patterns of activity in vivo, even if the frequencies are selected based on in vivo recordings. This is because it is unlikely that the volume of Hcrt cells stimulated by light will ever be activated synchronously. This was further supported by data showing that optical silencing of hcrt neurons promotes SWS (Tsunematsu et al. 2011, 2013).
These results were recently confirmed by Sasaki and collaborators (Sasaki et al. 2011), who used a chemogenetic approach called designer receptors exclusively activated by designer drugs (DREADDs) to activate and suppress hcrt neural activity. DREADD technology is based on the introduction of a mutated muscarinic receptor that is only activated in the presence of a synthetic ligand (clozapine N-oxide (CNO)). DREADDs allow bimodal modulation of neural activity with temporal resolution of several hours (Armbruster et al. 2007) and therefore is used as a complementary method of optogenetics in longer timescales. They found that activation of hcrt neural activity increased wakefulness, while suppression of hcrt activity promoted SWS.
We then showed that in sleep-deprived animals, optogenetic stimulation of Hcrt neurons failed to increase the probability of awakenings, suggesting that hcrt control of sleep–wake transitions is under the dependence of sleep homeostasis (Carter et al. 2009b). However, the effect of optogenetic stimulations of hcrt persisted in mice that are unable to synthesize histamine, suggesting that the histaminergic system is not required for the effect of hcrt on sleep-to-wake transitions. This effect does not rule out a possible role of the Hcrt–TMN connection in other features of sleep dynamics, as evidenced by the rescue of sleepiness in narcoleptic mice when replacing Hcrtr2. Finally, we showed that downstream arousal centers such as the LC neurons increased their activity (as measured by c-Fos expression) in response to hcrt optogenetic stimulation. Because previous work showed an excitatory effect of hcrt on LC-NE neurons (Bourgin et al. 2000), we investigated the hcrt–LC connection and focused our experimental investigations on the noradrenergic LC as a new target for optogenetic manipulation.
In a follow-up study, we genetically targeted LC-NE neurons by stereotaxic injection of a Cre recombinase-dependent adeno-associated virus (rAAV) into knock-in mice selectively expressing Cre in tyrosine hydroxylase (TH) neuron (Carter et al. 2010). We found that both NpHR and ChR2 were functional and could inhibit and activate, respectively, LC-NE neurons both in vitro and in vivo. We found that optogenetic low-frequency (1–10 Hz) stimulation of LC-NE neurons caused immediate (<5 s) sleep-to-wake transitions from both SWS sleep and REM sleep. Detailed time/frequency analysis revealed that 20 pulses delivered over 5 s were sufficient to induce an awakening (Carter et al. 2010). Stimulation of LC neurons during wakefulness increased locomotor activity and the total time spent awake, confirming the strong arousal effect. LC-NE stimulation can be so robust as to wake an animal from isoflurane Hypnotics (Vazey and Aston-Jones 2014). In contrast, NpHR-mediated silencing of LC-NE neurons decreased the duration of wake episodes but did not block sleep-to-wake transitions when animals were asleep. Taken together, this study showed that activation of LC-NE neurons is necessary for maintaining normal durations of wakefulness (NpHR experiment) and sufficient to induce immediate sleep-to-wake transitions, sustained wakefulness, and increased locomotor arousal. Thus, we proposed that the LC-NE neurons act as a fast tuning system to promote sleep-to-wake transitions and general arousal. Interestingly, we found that sustained optical activation of LC-NE neurons induces locomotor arrest (Carter et al. 2010), an effect likely caused by the depletion of norepinephrine from LC terminals or overexcitation of downstream motor nuclei. Such behavioral arrests share common symptoms with cataplexy, catatonia, or behavioral freezing both in animal models of narcolepsy and in human patients (Scammell et al. 2009). Thus, we hypothesized that behavioral arrests in narcoleptic mice could be caused by the lack of inhibitory control of LC neurons, possibly exerted under normal conditions by GABAergic neurons sensitive to Hcrt (and likely expressing HcrtR2) in the peri-LC region.
Most recently, we tested the hypothesis that LC activity gates hcrt neuron’s effects on sleep-to-wake transitions (Carter et al. 2012). We took a dual optogenetic approach to stimulate hcrt neurons while concomitantly inhibiting or stimulating noradrenergic LC neurons during SWS sleep. Silencing LC neurons during hcrt stimulation blocked hcrt-mediated sleep-to-wake transitions. To test whether an increase in LC excitability would facilitate Hcrt-induced awakenings, we used a variant channel rhodopsin known as step functional opsin (SFO). This channel can be activated by short pulses of blue light (1–10 ms) and can stay open up to 1 min, letting the flow of cations into the cell without necessarily reaching the depolarization threshold. Thus, when an SFO was expressed in LC neurons and LC neurons were primed with a 10-ms pulse, Hcrt stimulation was much more effective at eliciting sleep-to-wake transitions (Carter et al. 2012). Taken together, our results show that the LC serves as a necessary and sufficient downstream effector for hcrt-mediated SWS-to-wake transitions during the inactive period.
4 Hcrt and LC-NE System Dynamics
Across our experimental studies, we observed that optogenetic manipulation of hcrt and LC-NE neurons affects sleep-to-wake transitions with dramatically different temporal dynamics (Adamantidis et al. 2010; Carter et al. 2009b, 2010, 2012). Acute optical activation of hcrt neurons causes sleep-to-wake transitions over a time period of 10–30 s, while stimulation of LC neurons causes sleep-to-wake transitions in less than 5 s. The delayed effect of Hcrt on transitions may be explained by in vitro recordings of laterodorsal tegmental neurons in response to Hcrt, which show maximal depolarizations 20–30 s after bath application of Hcrt. Another explanation was provided by a mathematical conductance-based model of Hcrt and LC neurons (Carter et al. 2012; de Lecea and Huerta 2014). According to this model, Hcrt would slowly depolarize LC neurons’ subthreshold during a time window that is consistent with variable integration, that is, a “safety” window that would prevent spontaneous awakenings to irrelevant signals. If all conditions are met and the depolarization continues, then LC neurons produce spikes that may reach the awakening threshold.
One explanation is that hcrt neurons may act as an upstream integrator of arousal during hypothalamic-related functions, while the LC-NE system acts as a primary effector for arousal, stress, and attention (de Lecea and Huerta 2014). However, the neuronal effector systems are likely redundant and activated by distinct sets of inputs. Therefore, we cannot rule out that blocking other arousal systems, such as the central histaminergic and cholinergic systems, would also severely affect hcrt-induced behavioral state transitions in other experimental conditions.
Besides the short-term effects of photostimulation, it is of interest that experiments applying sustained ~1–4-h photostimulation of hcrt neurons showed increased sleep-to-wake transitions without changing the total duration of wakefulness (Carter et al. 2009b; Rolls et al. 2011), whereas long-term photostimulation of LC-NE neurons significantly increased wakefulness duration (Carter et al. 2010). It thus seems that Hcrt neurons are very sensitive to sleep pressure and do not elicit awakenings efficiently in conditions of sleep deprivation. Interestingly, Muhlethaler and colleagues have observed that the sensitivity of Hcrt neurons to noradrenergic innervation depends on sleep pressure (Grivel et al. 2005). Together, these results suggest that the hcrt system may regulate sleep–wake boundaries, while LC-NE neurons may rather control wake duration by increasing cortical membrane potential and desynchronizing the cortical EEG. Also, interestingly, we showed that control of the boundaries between sleep states is crucial for the consolidation of long-term memory (Rolls et al. 2011).
Obviously, the Hcrt–LC connection is not the only circuit involved in sleep-to-wake transitions. The role of histamine as a neuromodulator and powerful actor in sleep/wake cycles has long been documented. Anatomical studies revealed strong connectivity between lateral hypothalamic neurons and histaminergic cells in the tuberomammillary posterior hypothalamus . Trivedi et al. showed that His cells express Hcrtr2 (Trivedi et al. 1998). Moreover, Haas and colleagues demonstrated in slice experiments that His cells can be depolarized by Hcrt infusion (Eriksson et al. 2001). More recently, Burdakov and colleagues (Schone et al. 2014) have shown that optogenetic activation of Hcrtr2 is crucial for a reliable output of histaminergic neurons. These data, together with those of Mochizuki et al.’s discussed above, suggest an essential role of Hcrt-His circuitry in maintaining appropriate wakefulness. Thus, a picture is emerging in which the interaction of Hcrt with a network of neuromodulators defines the dynamic of sleep-to-wake transitions (Fig. 2). According to this scheme, Hcrt plays a fundamental role as a non-redundant neuromodulator of neuromodulators, providing the appropriate timescale of integration across multiple variables and transmitting this information into different timescales encoded by other transmitters such as norepinephrine, acetylcholine, or histamine.
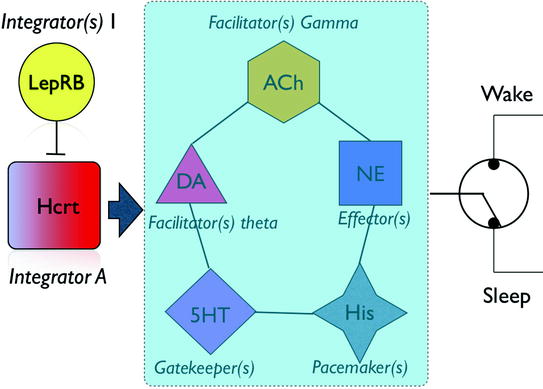
Fig. 2
Schematic of the interactions between Hcrt and arousal circuits. Hcrt neurons integrate information from metabolic, circadian, and limbic structures and convey the integrated information to a network of effectors, each of which has a different role in establishing the dynamic of a behavioral state transition
5 Concluding Remarks
As optogenetics unravels the role of individual transmitters in sleep/wake dynamics, it may be possible to build a larger-scale framework predictive of sleep architecture based on the activity of ensembles of neurons. Such a framework could have important consequences on the treatment of sleep disorders and other neuropsychiatric conditions in which arousal state transitions are impaired.
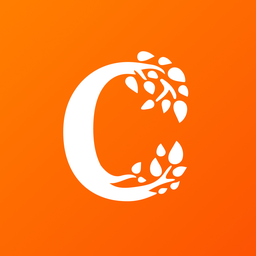
Full access? Get Clinical Tree
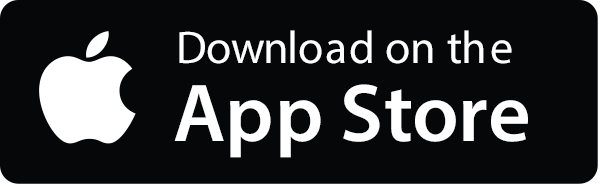
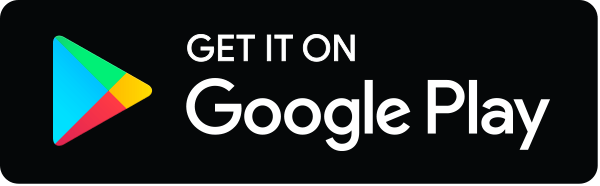