Fig. 1
Effects of sleep deprivation on plasticity-related gene expression. Symbols indicate different sleep deprivation methods, brain areas, targets of detection assays, and animal models used (respectively, polygons in red, green, blue, and orange). References listed at the bottom are referred as smaller numbers in parentheses (larger numbers or letters in parentheses refer to gene/protein family, while question marks indicate unspecified form, e.g., Homer1a, b or c)
2.1.5 Automated deprivation based on the computer analysis of neural and muscle signals
Sleep deprivation can be improved by the use of a feedback computer system for triggering cage movements depending on pre-established thresholds of neural and muscular activity (Ocampo-Garces et al. 2000; Vivaldi et al. 2008; Wu et al. 2011). In addition to the visual inspection of behavior, electrophysiological signals such as intracerebral local field potentials (LFPs) or cortical electroencephalogram (EEG) can be used to inform the experimenter about when to interfere with the animal. For instance, once the animal begins to sleep, the EEG is characterized by waves with higher amplitude and lower frequencies in comparison with the waking state; this slow-wave activity within the delta range (<4 Hz) is characteristic SWS. In the case of REM sleep, the presence of hippocampal theta rhythm (4–10 Hz) is one of the most reliable electrophysiological signals (Franken et al. 1991). The method is suitable for the use of yoked controls, i.e., animals recorded in parallel in which the perturbations occur at approximately the same times, but uncoupled from the wake–sleep states. Computer software can identify either SWS or REM and then prompt a mechanical perturbation of the animal, typically a sudden movement of the entire cage or recording box. Such automatic supervision is very precise and reliable, allowing for the use of yoked controls as explained above, but improved with the precise and instantaneous perturbations of both experimental and control animals (Rechtschaffen et al. 1999). The main disadvantage of the method is the more expensive and sophisticated setup.
2.2 Methods for the Assessment of Gene Expression and Protein Levels
2.2.1 In Situ Hybridization
This technique uses a complementary DNA or RNA strand to detect a certain sequence of mRNA or DNA in the tissue, which for instance may be an entire brain section. Labeling can be radioactive or antigen based, using fluorophores or chromophores (Gall and Pardue 1969; Jin and Lloyd 1997). The method is suitable for determining cell-specific and region-specific changes in gene expression directly on brain sections.
2.2.2 Reverse Transcriptase Quantitative Polymerase Chain Reaction (RT-qPCR)
One important variation of classical polymerase chain reaction (PCR) is quantitative PCR (qPCR), which precisely quantifies DNA sequences (Bustin et al. 2005). A second very important variation broadly used for gene expression detection is RTq-PCR. Instead of DNA, this method amplifies mRNA sequences using reverse transcriptase, polymerizing cDNA sequences as a product of the reaction. This method is very sensitive and also quantitative, allowing for the assessment of the amount of mRNA of a certain gene even if it is expressed at very low levels (Shiao 2003; Bustin et al. 2005); however, the method is performed on tissue homogenates and therefore allows for less regional specificity.
2.2.3 DNA Hybridization Array (Microarray)
This method uses a plate with multiple spots that contain different DNA sequences for genes of interest. These sequences are hybridized with cDNA or cRNA extracted from a tissue sample. Hybridization levels can be detected by chemiluminescence or by the use of fluorophores. Typically, an important step before hybridization is the labeling of target sequences of the sample; mRNA is purified and submitted to RT-PCR to synthesize cDNA, which is labeled afterward with a fluorescent component (Freeman et al. 2000). The main advantage of this method is the ability to assess a very large number of genes for a single tissue sample.
2.2.4 Western Blotting
This method is used to detect and sort specific proteins in tissue homogenates using gel electrophoresis. After being separated, proteins are transferred to a membrane (e.g., nitrocellulose), treated with a protein-rich blocking reagent to avoid non-specific labeling, incubated with a primary antibody (IgG) to detect a specific protein of interest, washed and then incubated with a secondary antibody (anti-IgG) associated with a reporter molecule (e.g., horseradish peroxidase or alkaline phosphatase). Detection is performed using a chromogenic or luminescent substrate (Gallagher et al. 2004). This method detects proteins and therefore provides only an indirect measurement of gene expression.
2.2.5 Immunohistochemistry
This method uses the same principle of antibody detection described above, but in tissue sections instead of homogenate samples (Jin and Lloyd 1997). This method also detects proteins and therefore provides only an indirect measurement of gene expression.
3 Sleep Deprivation and Gene Expression
Some of the most important effects of sleep deprivation are modifications in gene expression, which can reflect the activation or suppression of cellular processes leading to changes in neuronal function and synaptic connections. The analysis of gene expression changes provides therefore a privileged window into brain function. As described in Sect. 2, there are different methods for the sleep deprivation of animal and many ways to measure changes in gene expression profiles. Most of the studies on sleep deprivation that aim to analyze gene expression are performed in rodents (Pompeiano et al. 1992, 1997; Cirelli et al. 1995, 2006; Maloney et al. 2002; Cirelli 2006; Terao et al. 2006), but there are also important studies in flies (Wang et al. 2010), birds (Jones et al. 2007), and fish (Appelbaum et al. 2010). Sleep deprivation studies are often difficult to compare, due to the use of different deprivation methods, durations, and animal models. There is also considerable variation in the methods employed to quantify gene expression levels. For instance, the decision to measure protein or mRNA may bias toward different outcomes, depending on the timescale of the experiment. Since mRNA transcriptional regulation precedes protein synthesis, experimental designs must carefully consider whether the method of choice for gene expression assessment is compatible with the temporal windows chosen.
Most of the gene expression studies use the microarray method, which allows for the simultaneous measurement of large numbers of genes [from 10,000 transcripts (Cirelli and Tononi 2000a, b, c) to more than 26,000 (Cirelli et al. 2006)]. Such screens reveal that sleep deprivation causes major changes in gene expression not only in the brain but also in the periphery. Stress response genes are frequently targeted in sleep deprivation experiments, since many methods of sleep deprivation are considered stressful due to the use of unpleasant stimuli (e.g., disk-over-water method). Sleep deprivation per se may be considered a stressful situation, irrespective of which deprivation method is used. Under this assumption, sleep loss is expected to always trigger a stress response.
Several genes expressed during spontaneous waking have their expression upregulated in the central nervous system during sleep deprivation (Cirelli et al. 2006). These genes comprise immediate early genes that encode transcription factors, growth factors, adhesion molecules, neurotransmitter receptors and transporters, and enzymes (Cirelli and Tononi 2000a, b, c). A gene screen study (Cirelli and Tononi 2000a, b, c) showed a wide range of genes whose expression is upregulated by sleep deprivation: the activity-regulated cytoskeleton-associated protein (Arc), the C/EBP homology protein (CHOP), the immediate early response 5 (IER5), the nerve growth factor-induced protein A (NGFI–A, homologue of Zif–268, Krox–24, Egr–1, and ZENK), the nerve growth factor-induced protein I–B (NGFI–B, also known as Nr4a1), the neuroblastoma ras oncogene (N–Ras), the signal transducer and activator of transcription (3Stat3), the glucose type I transporter (Glut1), the VGF nerve growth factor inducible (Vgf), brain-derived neurotrophic factor (BDNF), the tyrosine-related kinase B (TrkB, also known as NTRK2), the coagulation factor III (F3), the binding immunoglobulin protein (BiP, also known as HSPA5 or HSP70), the endoplasmic reticulum resident protein 72 (ERP72, also known as PDIA4), the 75-kDa glucose-regulated protein (GRP75, also known as HSPA9), the 60-kDa heat-shock protein (HSP60), the 70-kDa heat-shock protein (HSP70), chromogranin C, synaptotagmin IV, the adrenergic receptors α and β, the GABA receptor B, the glutamate NMDA receptors 1A, 2A, and 3A, the glutamate AMPA receptors GluR2 and GluR3, nicotinic acetylcholine receptor B, the thyroid hormone receptor beta (TRb), the glutamate/aspartate transporter (GLAST), and the Na/Cl transporter NTT4/Rxt1. Also upregulated are the enzymes aryl sulfotransferase, c-jun N-terminal kinase 1, serum/glucocorticoid-induced serine/threonine kinase, calmodulin, cyclin D2, LIM domain only protein 4 (LMO-4), and metallothionein 3. All these genes were systematically investigated through mRNA differential display and cDNA microarrays, being upregulated in rats deprived of sleep for 8 h (Cirelli and Tononi 2000a, b, c). Another study from the same research group assessed the long-term effects of sleep deprivation on mRNA levels in the rat cerebral cortex. After 1 week of sleep deprivation, 75 transcripts had their levels increased in comparison with the levels found in spontaneously awake animals, as well as animals deprived of sleep for a few hours. These transcripts comprised of mRNA for immunoglobulins, stress response proteins, minoxidil sulfotransferase, globins, and cortistatin. Altogether, these results indicate that sleep deprivation induces marked inflammatory and stress responses in the brain (Cirelli et al. 2006).
4 Sleep Deprivation and Early Changes in Gene Expression
Sleep deprivation upregulates the expression of several immediate early genes, as well as alternative splice variants induced by neuronal activity, whose protein products are involved in regulating a wide variety of cellular processes that ultimately influence neuronal plasticity and brain function (Tischmeyer and Grimm 1999).
4.1 c-fos
The immediate early gene c-fos gives rise to the fos protein that acts as a rather general transcription factor. Expression of the fos gene is upregulated by membrane depolarization and therefore widely used as a general marker of neuronal activity (Kovacs 1998; Cirelli and Tononi 2000a, b, c). The expression of c-fos is upregulated in several brain areas by spontaneous wakefulness (Pompeiano et al. 1994) as well as forced sleep deprivation (Cirelli et al. 1995). Both the mRNA and the protein levels of c-fos are elevated in rats when the sleep cycle is arrested, with a wide anatomical distribution that comprises the cerebral cortex, portions of the hypothalamus, the medial preoptic area, thalamic nuclei, and nuclei of the dorsal pontine tegmentum (Cirelli et al. 1995). Somewhat similar results were obtained in cats using immunohistochemistry, which revealed increased c-fos levels in the preoptic area and lateral hypothalamus of sleep-deprived animals (Ledoux et al. 1996). In mice subjected to sleep deprivation for 6h, c-fos mRNA protein levels were increased in the cerebral cortex, basal forebrain, thalamus, and cerebellum (Terao et al. 2003). A study of sleep deprivation in neonate rats found increased c-fos mRNA levels in the cerebral cortex and hippocampus at postnatal days 16, 20, and 24, with a return to basal levels after a 2-h recovery sleep period (Hairston 2004).
An anatomically comprehensive study of sleep-deprived mice used laser microdissection and cDNA microarrays to show upregulation of c-fos mRNA levels in visual and caudal somatosensory cortex, agranular insular cortex, orbitofrontal cortex, piriform cortex, amygdala, dorsal caudate putamen, and cerebellum, with downregulation in the ventral posteromedial nucleus of the thalamus. C-fos expression was largely coincident with expression of the excitatory neuron-specific vesicular glutamate transporter 1 (Slc17a7) and overlapped with Arc and Nr4a1 expression in visual and agranular insular cortices (Thompson 2010).
Sleep deprivation of non-mammalian vertebrates by and large yields similar results. In the white-crowned sparrow, c-fos mRNA levels are increased in several portions of the telencephalon after 6 h of sleep deprivation (Jones et al. 2007). In the zebra fish, sleep deprivation for 3 h or 6 h leads to c-fos mRNA upregulation in the olfactory bulb, telencephalon, diencephalon, and rhombencephalon, with a return to basal levels after recovery sleep (Appelbaum et al. 2010).
4.2 Zif-268
The transcription factor Zif-268 (zinc finger protein 268) is also known as NGFI-A (nerve growth factor-induced protein A), EGR-1 (early growth response protein 1), Krox-24, and ZENK (acronym of the initial letters of Zif-268, Egr-1, NGFI-A, and Krox-24). The Zif-268 protein regulates the expression of many genes involved with plasticity, mitogenesis, and differentiation, including synapsins I and II, and synaptobrevin II (Knapska and Kaczmarek 2004). Zif-268 was found to be upregulated after 6 h of sleep deprivation in the rat cerebral cortex assessed through Northern blotting (O’Hara et al. 1993). In rats submitted to sleep deprivation for up to 10 days using the disk-over-water method (Landis et al. 1993), immunocytochemistry and Northern blotting showed elevated Zif-268 expression in the dorsal raphe, lateral habenula, superior colliculus, and ventral periaqueductal gray. These neuroanatomical locations were not corroborated by a subsequent study, which applied in situ hybridization to brains of animals subjected to sleep deprivation by gentle handling for 3, 6, 12, and 24 h (Pompeiano et al. 1997). Increased mRNA levels were found in the cerebral cortex and caudate–putamen (Pompeiano et al. 1997). The same authors found that 3 h of sleep deprivation was associated with increased Zif-268 levels in the cerebral cortex but not in the locus coeruleus, while 12 or 24 h of sleep deprivation induced Zif-268 expression in the locus coeruleus, the parabrachial nuclei, and the superior and inferior colliculi (Tononi et al. 1994). A plausible explanation for the somewhat divergent findings may reside in the different amounts of sleep deprivation applied. Longer periods of deprivation may trigger self-regulation mechanisms such as the negative feedback enabled by the Zif-268 response, in which the protein recognizes consensus sequences in the promoter region of the gene, allowing for downregulation of its own expression (Christy and Nathans 1989).
More recently, a study in mice showed that sleep deprivation for 6 h followed by a recovery period of 4 h leads to the upregulation of Zif-268 mRNA levels in the cerebral cortex and basal forebrain (Terao et al. 2003). The deprivation-related increase of Zif-268 levels seems to obey a very similar dynamics in the avian brain. In white-crowned sparrows, telencephalic Zif-268 mRNA levels were reported to increase 117 % after 6 h of sleep deprivation, with a 90 % increase from spontaneous waking to control (Jones et al. 2008).
4.3 Arc
Another immediate early gene involved in neuronal plasticity and affected by sleep deprivation is Arc (also known as Arg3.1), encoded by mRNA transported to post-synaptic dendritic terminals for local translation (Lyford et al. 1995). Arc participates in synaptic remodeling by regulating the dynamics of F-actin, AMPA receptors, and Ca2+/calmodulin-dependent protein kinase II (CaMKII) (Bramham et al. 2010; Okuno et al. 2012).
Arc levels are upregulated in rats after both acute (8 h) (Cirelli and Tononi 2000a, b, c) and chronic (1-week) sleep deprivation, when assessed by microarrays and confirmed by real-time quantitative polymerase chain reaction (RT-qPCR) and RNase protection assay (RPA) (Cirelli et al. 2006). Another study found Arc mRNA levels to be upregulated in the cerebral cortex of mice and rats subjected to sleep deprivation for 6 h (Terao et al. 2006). Similar results were obtained in the cerebral cortex and hippocampus after 8 h of sleep deprivation in rats, with recovery to basal Arc mRNA levels after 2 h of sleep (Taishi et al. 2001). Corroborating these findings, a study with C57BL/6J mice showed higher levels of Arc mRNA in the hippocampus, amygdala, neocortex, and piriform cortex of sleep-deprived animals, in comparison with controls. However, increased Arc levels were detected even after a 4-h sleep rebound period (Thompson 2010). Another study in rats using gene chip analysis and 24 h of sleep deprivation by gentle handling found a 1.2-fold decrease of Arc mRNA levels in the hypothalamus and a twofold increase in the hippocampus, in comparison with controls (Conti et al. 2006). A meta-analysis of datasets comprising 91 different genes found Arc mRNA to be upregulated in the cerebral cortex, hippocampus, olfactory bulb, and striatum of mice deprived of sleep for 6 h (Wang et al. 2010). Similar effects occur in the brain of the white-crowned sparrow, with a 66 % increase in Arc mRNA levels after 6 h of sleep deprivation (Jones et al. 2008).
4.4 Homer
Another gene of interest is Homer1, which is widely expressed in the brain and other tissues with two main splice variants: a short form called Homer1a, which is induced by neuronal activity, and long forms called Homer1b and c, which have constitutive expression (Shiraishi-Yamaguchi and Furuichi 2007). Homer1a inhibits the scaffolding action of the long forms, decreasing glutamatergic signaling and diminishing dendritic spines (Tu et al. 1998). Like Arc (Sect. 4.3), Homer1a is broadly upregulated in the brains of sleep-deprived rats (Cirelli et al. 2006; Maret et al. 2007). Additionally, it is expressed in spontaneously awake rats, which suggests that Homer1a is generally associated with wakefulness and neuronal activity (Thompson 2010). Homer1a mRNA is upregulated in the somatosensory cortex of rats deprived of sleep for 6 h during the day (sleep period); in contrast, Homer1a gene expression is downregulated by sleep deprivation during the evening (active period); similar results of a lesser magnitude were obtained for Homer1bc (Nelson et al. 2004). Homer1a is significantly overexpressed in the cerebral cortex, hippocampus, and striatum in three different mouse genotypes (Maret et al. 2007). Another study using microarray analysis and the disk-over-water method presented compatible results: Rats deprived of sleep for 8h showed upregulated Homer1a levels in comparison with animals allowed to sleep, while sleep deprivation for 7 days yielded a non-significant tendency for transcriptional downregulation related to short-term sleep deprivation (8 h) and spontaneous waking (Cirelli et al. 2006). Homer1 upregulation was detected in the basal forebrain and cerebral cortex of rats submitted to sleep deprivation by introducing novel objects and tapping the cage during 6 h (Terao et al. 2006). Another study used in situ hybridization to detect Homer1 upregulation in rats deprived of sleep for 24 h (Conti et al. 2006). An investigation of Homer1 expression after 3, 6, 9, and 12 h of sleep deprivation found gene upregulation in the cerebral cortex for all these periods (Mackiewicz et al. 2007). Although these particular three studies (Conti et al. 2006; Terao et al. 2006; Mackiewicz et al. 2007) did not specify which Homer1 variation was measured, it is likely that it was Homer1a, since most of the other studies cited in this section specifically indicate Homer1a to be upregulated by sleep deprivation (Mackiewicz et al. 2007). A meta-analysis found Homer1a levels to be upregulated in mice after 6 h of sleep deprivation in the cerebral cortex, hippocampus, olfactory bulb, and striatum (Wang et al. 2010). Interestingly, experiments performed in drosophila showed Homer downregulation in sleep-deprived flies. Homer1a uncouples the metabotropic glutamate receptor from other Homer isoforms. Drosophila has only one Homer isoform, which acts in the opposite direction of Homer1a (Zimmerman et al. 2006; Mackiewicz et al. 2008).
4.5 Nr4a
Gene expression is highly influenced by nuclear receptors that act as transcription factors upon activation by steroid and thyroid hormones, among other ligands (Green and Chambon 1988; McKenna and O’Malley 2002). Some nuclear receptors are regulated as immediate early genes, such as the NR4A subfamily of orphan receptors that include the nerve growth factor IB (NGFIB/NR4A1), the nuclear receptor-related protein 1 (NURR1/NR4A2), and the neuron-derived orphan receptor 1 (NR4A3/NOR1) (Maxwell and Muscat 2006; Hawk and Abel 2011). Sleep deprivation affects Nr4a expression, which is activated by the same calcium-dependent factors that upregulate the memory-related IEG expression reviewed above (Hawk and Abel 2010). In 6-h sleep-deprived rats, Nr4a mRNA levels were found to be upregulated in the cerebral cortex, basal forebrain, and hypothalamus (Terao et al. 2006). Another study using gene chip analysis found a 100 % increase in Nr4a3 mRNA expression in the rat hypothalamus after 24 h of sleep deprivation (Conti et al. 2007). In white-crowned sparrows deprived of sleep for 6 h, Nr4a1 mRNA levels in the telencephalon were found to be increased by 60 %, in comparison with control animals allowed to sleep (Jones et al. 2008). An assessment of Nr4a1 gene expression in mice deprived of sleep for 6h using laser microdissection and microarray hybridization found elevated mRNA levels in the amygdala and in the primary visual, agranular insular, orbitofrontal and piriform cortices, as well as in the cerebellum and putamen; controls allowed to sleep showed increased levels of Nr4a1 and Arc in the rostral and ventral caudate–putamen, as well as in the motor and rostral somatosensory cortices; sleep-deprived mice showed Nr4a1 upregulation in the caudal dorsolateral putamen, overall a very similar profile found for Arc expression. (Thompson 2010). A meta-analysis of microarray data in mouse, rat, sparrow, and fly identified the Nr4a gene family as conserved genes affected by sleep deprivation (Wang et al. 2010).
The upregulation of immediate early gene expression by sleep deprivation requires the involvement of secondary messengers. An investigation of the levels of phosphorylated cAMP response element-binding protein (p-CREB) after 3 h of sleep deprivation detected a significant and widespread increase in the cerebral cortex (Cirelli and Tononi 2000a, b, c). Another study found the mRNA levels of CaMKII to be upregulated in the cerebral cortex and basal forebrain of rats deprived of sleep for 6 h (Terao et al. 2006). In contrast, other researchers found that 48 h of sleep deprivation reduces CREB mRNA levels to the levels found in controls kept in their home cages; similar results were obtained for CaMKII (Guzman-Marin 2006). Chemical lesions of the noradrenergic system showed that the expression of Zif-268 and c-fos during wakefulness depends critically on the integrity of the locus coeruleus (Cirelli et al. 1996). The implication of this finding is that calcium-dependent immediate early gene expression, and presumably upstream events such as increases in cAMP and p-CREB levels, also requires noradrenergic modulation.
5 Sleep Deprivation and Neurotrophic Factors
The gene expression and protein levels of neurotrophic factors involved with cell growth and function (Boyd and Gordon 2003a, b) have also been shown to be affected by sleep deprivation (Cirelli and Tononi 2000a, b, c; Sei et al. 2000; Fujihara et al. 2003; Hamatake et al. 2011; Ventskovska et al. 2014; Wallingford et al. 2014; Zielinski et al. 2014). Most studies were concerned with BDNF, a neurotrophin of great importance for neuronal plasticity and survival (Ghosh et al. 1994; Ventimiglia et al. 1995; Lipsky and Marini 2007; Sossin and Barker 2007). A study of rats selectively deprived of REM sleep for 6 h found BDNF protein levels to be unchanged in the hippocampus and decreased in the cerebellum and brain stem (Sei et al. 2000). A lack of hippocampal change in BDNF mRNA levels after sleep deprivation was also reported in rats subjected to full sleep deprivation for 8 h; in contrast, the same animals showed a significant increase in BDNF mRNA levels in the cerebral cortex (Taishi et al. 2001). Rats subjected to sleep deprivation (for 8 and 48 h) by an intermittent treadmill method showed a significant decrease of mRNA and protein BDNF levels in the hippocampus, but not in the cerebral cortex (Guzman-Marin 2006). A different study of sleep deprivation in rats detected a significant increase of BDNF mRNA levels in the cerebral cortex (Cirelli and Tononi 2000a, b, c). Interestingly, BDNF mRNA levels were significantly increased in the cerebral cortex when animals were sleep deprived for 8 h by introducing new objects in the cage, but not in animals deprived of sleep for 1 week using the disk-over-water method (Cirelli et al. 2006). While this comparison is imperfect, varying both the duration and the method for sleep deprivation, it suggests that deprivation by cognitive challenge may impact gene expression quite differently from deprivation due to non-cognitive physiological challenge.
An investigation of sleep deprivation using gene chip methodology and in situ hybridization found BDNF mRNA levels to be upregulated in the piriform cortex, dorsal endopiriform nucleus, and hippocampus (Conti et al. 2007). Another study used microarrays and RT-PCR to show that BDNF mRNA levels are increased in the rat cerebral cortex after 96 h of REM sleep deprivation, with a return to basal levels after 24 h of sleep recovery (Guindalini et al. 2009). In neonate rats deprived of sleep for up to 180 min, mRNA BDNF levels were increased in the cerebral cortex at postnatal days P20 and P24 (Hairston 2004). Interpretation of the somewhat divergent results is complicated by differences in the methods employed for sleep deprivation, (e.g., the introduction of new objects versus intermittent locomotion on a treadmill), in the duration of the deprivation protocols, in brain region analyzed, and in the age of the animals.
If the corpus of published studies on BDNF and sleep deprivation fails to systematically cover the parameter space, investigation of other neurotrophins is even sparser. Sei et al. compared the effects of 6h of REM sleep deprivation on BDNF and nerve growth factor (NGF) protein levels in the rat hippocampus, cerebellum, and brainstem. NGF levels were significantly decreased in the hippocampus, but were unchanged in the cerebellum and brainstem (Sei et al. 2000). Another study showed that 6h of total sleep deprivation was associated with a significant increase in the number of NGF-immunoreactive cortical neurons in the mice barrel field (Brandt et al. 2001). An investigation of the diurnal variations of neurotrophin-3 (NT-3) and BDNF levels in several brain regions of juvenile rats (2 weeks) detected increased BDNF levels in the dark phase and decreased levels in the light phase. NT-3 levels showed the opposite profile in the neocortex (Hamatake et al. 2011). Another study observed that intracerebroventricular injections of NT-3 and neurotrophin-4 (NT-4) in adult rabbits promote non-REM sleep in a dose-dependent manner (Kushikata et al. 2003). A great deal of additional experimentation is needed to elucidate the effect the sleep deprivation on neurotrophin expression.
6 Sleep Deprivation Changes Expression of Genes Related to Protein Synthesis
A microarray study of mice subjected to sleep deprivation for 5 h detected alterations in the hippocampal expression of 533 genes (Vecsey et al. 2012). A bioinformatics assessment pointed to a marked downregulation of translation through the insulin signaling pathway, including the mammalian target of rapamycin (mTOR), a serine/threonine protein kinase with a major role in cell metabolism and protein synthesis (Wullschleger et al. 2006). Sleep deprivation decreased both the absolute and the phosphorylated levels of mTOR, with a return to basal levels of expression after a 2.5-h period of sleep. The result suggests that the physiological deficits caused by sleep deprivation are in part related to the impairment of protein synthesis mediated by mTOR.
There is also evidence that sleep deprivation increases the expression of molecular chaperones involved with the folding and oligomerization of newly synthesized proteins. For instance, brain expression of the binding immunoglobulin protein (BiP), also known as the 78-kDa glucose-regulated protein (GRP-78), is significantly upregulated by sleep deprivation in drosophila (Shaw et al. 2000), birds, and rodents deprivation (Cirelli and Tononi 2000a, b, c; Shaw et al. 2000; Greenspan et al. 2001; Naidoo et al. 2005; Cirelli et al. 2006; Mackiewicz et al. 2007; Jones et al. 2008). Found in the endoplasmic reticulum, BiP is a chaperone that binds to newly synthesized proteins to facilitate their folding and oligomerization. The upregulation of BiP expression by sleep deprivation likely determines the upregulation of protein levels corresponding to a plethora of different genes.
7 Changes in Serum Protein Levels After Sleep Deprivation may Indirectly Reflect Changes in Gene Expression
Given the important influence of sleep on a great number of metabolic pathways, it is not surprising that sleep deprivation induces major metabolic disarray, including changes in serum protein levels. These may reflect changes in gene expression, but may also reflect changes in the release, degradation, and turnover of these molecules. The hormones, cytokines, and other proteins whose expression levels are affected include leptin, ghrelin, the thyroid hormones T3 and T4, interleukin 6 (IL-6), TNF-alpha, and C-reactive protein (CRP). Combined, these changes lead to energetic imbalance, impairment of the glucose metabolism, and metabolic syndrome (Mullington et al. 2009). In particular, REM sleep deprivation increases IL-1B, IL-6, and IL-12 (P70) serum levels, as well as the liver expression of IL-1B and IL-6 receptors in rodents (Gorczynski et al. 2005; Pandey and Kar 2011). These findings corroborate and greatly extend the earlier demonstration of a modulatory role of sleep on the expression of interleukin-1 beta (IL-1B), an important pro-inflammatory cytokine (Mackiewicz et al. 1996). REM sleep deprivation also elevates the metabolic rate by increasing expression of the UCP1 gene, which encodes a protein involved in the dissipation of mitochondrial energy as heat, instead of ATP formation (Koban and Swinson 2005). An investigation of the effects of sleep deprivation on the inflammatory response in mice detected increased levels of IL-6 and IL-10 and decreased levels of TNF-alpha in animals treated with lipopolysaccharide (Weil et al. 2009).
In humans, one night of sleep loss leads to increased levels of IL-6 and TNF-alpha (Irwin et al. 2006). Chronic sleep loss for 1 week in young adults leads to increased levels of IL-6 in both males and females, while TNF-alpha is increased only in men (Vgontzas et al. 2004). Subjects with sleep limited to 4 h per night show increased levels of IL-6, in comparison with subjects sleeping 8 h per night; no significant differences between these groups were seen for TNF or CRP (Haack et al. 2007). Sleep restriction for 5 nights induces lymphocyte activation and upregulation of IL-1, IL-6, IL-17, and CRP (van Leeuwen et al. 2009). The studies of patients with obstructive sleep apnea are not conclusive: While some report increases, other find unchanged levels (Arnardottir et al. 2009). An investigation of 70 patients found that poor sleep is associated with higher concentrations of vascular endothelial growth factor (VEGF) and that fatigue-related reduction in activity is associated with increased TNF-alpha levels (Guess et al. 2009). Night-shift workers show increased levels of IL-6 and CRP, as well as elevated counts of white blood cells, neutrophils, lymphocytes, and platelets (Khosro et al. 2011). Overall, sleep deprivation is associated with a strong inflammatory response.
8 Sleep Deprivation and Genes Related to Cellular Stress Responses
Sleep loss stresses the organism as a whole, challenging its equilibrium at multiple levels, from the molecular and cellular domains to the complex interaction of entire systems, leading to behaviors such as anxiety, fear, aggressiveness, and helplessness (Bergmann et al.). Autonomic responses and activation of the hypothalamus–pituitary–adrenal (HPA) axis (Herman and Cullinan 1997; Ziegler and Herman 2002), including enhanced glucocorticoid release, lead to the transcriptional upregulation of specific genes. The mRNA levels of the serum/glucocorticoid-induced serine/threonine kinase 1 (Sgk1), which activates ion channels in response to changes in cell hydration, are increased after 8 h of sleep deprivation, but not after spontaneous waking. Sleep deprivation was achieved by multimodal sensory stimulation, which consisted of the presentation of novel objects or odors, moving the animal to another cage and tapping the cage (Cirelli and Tononi 2000a, b, c). Another study found that Sgk1 mRNA levels are increased almost twofold in the dorsal raphe nucleus and more than twofold in the locus coeruleus in rats deprived of sleep for 24 h. In this case, sleep deprivation was performed by gently handling the animal, and differential analysis of gene expression was used to assess mRNA levels (Conti et al. 2007). More recently, several stress-related genes including Sgk, the cyclin-dependent kinase inhibitor 1A (Cdkn1a), the brain-derived neurotrophic factor (BDNF), secretogranin II (Scg2), prokineticin 2 (Prok2), and the heat-shock 27-kDa protein 1 (Hspb1) were shown to be upregulated in animals kept awake for 6 h by multimodal sensory stimulation (including cage rotation). To assess gene expression, seven brain regions were submitted to laser microdissection, and main genes were selected through cDNA microarray for subsequent in situ hybridization confirmation (Thompson 2010).
Sleep deprivation also affects the expression of genes related to stress in glial cells, such as the macrophage inhibitor factor-related protein 14 (MRP14), the heat-shock protein 27 (Hsp27), and the aB-crystallin (Cryab) (Cirelli et al. 2006). MRP14 is found in microglial cells (Postler et al. 1997; Staba et al. 2002), while Hsp27 and Cryab are heat-shock proteins with a protective role in oligodendrocytes and astrocytes (Goldbaum and Richter-Landsberg 2001). Altogether, these results point to the global reach of sleep deprivation, which negatively affects the organism as a whole, while at the same time stressing individual cells quite locally.
9 Sleep Deprivation, Gene Expression, and Cognition
Sleep deprivation affects the expression of immediate early genes, genes coding for neurotrophic factors, and various other genes that directly or indirectly affect neuronal plasticity, which in turn support brain function and cognitive processes. However, the finding that many of these genes are up-regulated after sleep deprivation seems at odds with the established fact that sleep deprivation causes impairments in cognitive function. The fact that plasticity factors involved with learning and memory (Guzowski et al. 2000; Jones et al. 2001; Bozon et al. 2003) are up-regulated by sleep deprivation poses a conundrum, given the evidence that sleep deprivation produces mnemonic impairment (Pearlman 1969; Graves et al. 2003; Seugnet et al. 2011; Havekes et al. 2012; Kumar and Jha 2012). One possible explanation for these results is the notion that learning triggers gene expression in specific subsets of neurons, while sleep deprivation affects neuronal populations in a generalized manner. Such a scenario is compatible with evidence that REM sleep triggers the upregulation of Zif-268 and Arc expression in the cerebral cortex and hippocampus of rats previously exposed to novel waking experience (Ribeiro et al. 1999, 2002, 2007; Ulloor and Datta 2005). This view is also supported by results showing that the detrimental effects of sleep deprivation on contextual fear conditioning are caused by a disruption of signaling via cyclic adenosine monophosphate (cAMP) and protein kinase A (PKA). Sleep deprivation for 5 h was followed by electrophysiological recordings and stimulation of hippocampal slices in mice with or without exposure to contextual fear conditioning. Two forms of long-term potentiation (LTP) dependent on cAMP and PKA were impaired by sleep deprivation, while LTP forms independent of cAMP/ PKA signaling remained unaltered. Sleep deprivation decreased CREB phosphorylation required for Zif-268 transcriptional regulation, seemingly at variance with several studies showing increased Zif-268 expression after sleep deprivation (Sect. 4.2). Further experiments showed that inhibitors of cyclic nucleotide phosphodiesterases (PDE) rescued cAMP-dependent plasticity and cAMP levels in sleep-deprived mice. PDE-specific cAMP breakdown was enhanced in sleep-deprived mice, and in vivo PDE inhibition rescued the mnemonic deficit produced by sleep deprivation (Vecsey et al. 2009).
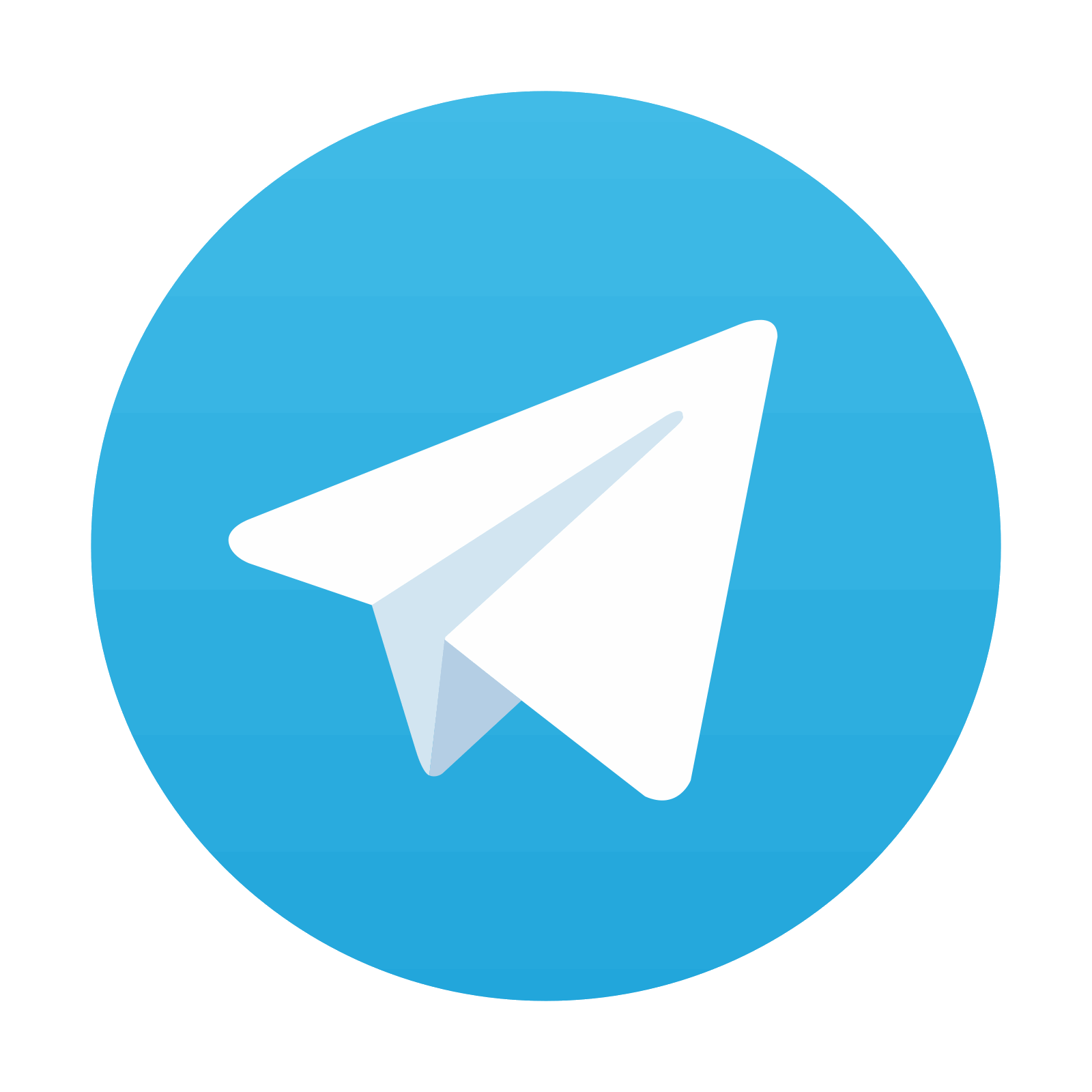
Stay updated, free articles. Join our Telegram channel
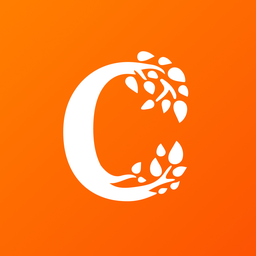
Full access? Get Clinical Tree
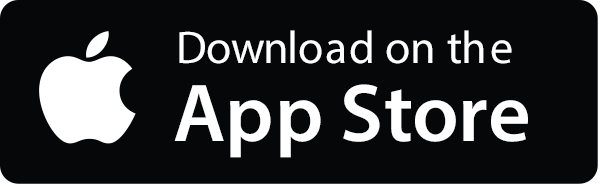
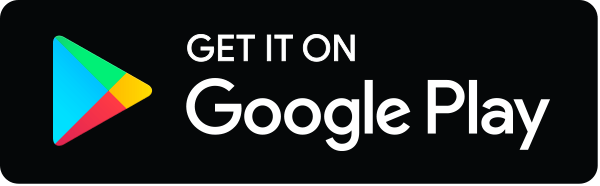