Differentiation and Survival of Nerve Cells
Radial Glial Cells Serve As Neural Progenitors and Structural Scaffolds
Neuronal Migration Establishes the Layered Organization of the Cerebral Cortex
Central Neurons Migrate Along Glial Cells and Axons to Reach Their Final Settling Position
Neural Crest Cell Migration in the Peripheral Nervous System Does Not Rely on Scaffolding
The Neurotransmitter Phenotype of a Neuron Is Plastic
The Transmitter Phenotype of a Peripheral Neuron Is Influenced by Signals from the Neuronal Target
The Transmitter Phenotype of a Central Neuron Is Controlled by Transcription Factors
The Survival of a Neuron Is Regulated by Neurotrophic Signals from the Neuron’s Target
The Neurotrophic Factor Hypothesis Was Confirmed by the Discovery of Nerve Growth Factor
Neurotrophic Factors Suppress a Latent Death Program in Cells
IN THE PRECEDING CHAPTER WE DESCRIBED how local inductive signals pattern the neural tube and establish the early regional subdivisions of the nervous system—the spinal cord, hindbrain, midbrain, and forebrain. Here we turn to the issue of how progenitor cells within these regions differentiate into neurons and glial cells, the two major cell types that populate the nervous system.
We discuss some of the molecules that specify neuronal and glial cell fates and how they are regulated. The basic mechanisms of neurogenesis endow cells with common neuronal properties, features that are largely independent of the region of the nervous system in which they are generated or the specific functions they perform. We also discuss the mechanisms by which developing neurons express neurotransmitters and synaptic receptors.
After the identity and functional properties of the neuron have begun to emerge, additional developmental processes determine whether the neuron will live or die. Remarkably, approximately half of the neurons generated in the mammalian nervous system are lost through programmed cell death. We examine the factors that regulate the survival of neurons and the possible benefits of widespread neuronal loss. Finally, we describe the existence of a core biochemical pathway that programs the death of nerve cells.
The Proliferation of Neural Progenitor Cells Involves Symmetric and Asymmetric Modes of Cell Division
The mature brain comprises billions of nerve cells and even more glial cells. Yet its precursor, the neural plate, initially comprises only a few hundred cells. From this simple comparison we infer that regulation of the proliferation of neural cells is a major driving force in shaping brain development. Histologists in the late 19th century showed that neural epithelial cells close to the ventricular lumen of the embryonic brain exhibit features of mitosis, and we now know that the proliferative zones surrounding the ventricles are the major regions involved in the production of neural cells in the cerebral cortex as well as other regions of the central nervous system.
At early stages of embryonic development most progenitor cells in the ventricular zone of the neural tube proliferate rapidly. Many of these early neural progenitors have the properties of stem cells: They can generate additional copies of themselves, a process called self-renewal, and also give rise to differentiated neurons and glial cells (Figure 53-1B). As with other types of stem cells, neural progenitor cells undergo stereotyped programs of cell division.
Figure 53-1 Neural progenitor cells have different modes of division.
A. Temporal sequence of neurogenesis in the mouse cerebral cortex. Neurons begin to accumulate in the cortical plate (CP) during the last 5 days of embryonic development. Within the cortical plate neurons populate the deep layers before settling in the superficial layers. (MZ, marginal zone; PP, preplate; SP, subplate; IZ, intermediate zone; SVZ, subventricular zone; VZ, ventricular zone; WM, white matter.)
B. Asymmetric and symmetric modes of cell division. A progenitor cell (P) undergoes asymmetric division to generate a neuron (N) and a glial cell (G) (left). A progenitor cell undergoes asymmetric division, giving rise to another progenitor cell and a neuron. This mode of division contributes to the generation of neurons at early stages of development, and of glial cells at later stages, typical of many regions of the central nervous system (middle). A progenitor cell undergoes symmetric division to generate two additional progenitor cells (right).
C. Time-lapse cinematography captures the divisions and differentiation of isolated cortical progenitor cells in the rodent. Lineage diagrams illustrate cells that undergo predominantly asymmetric division, giving rise to neurons (left), or symmetric division that gives rise to oligodendrocytes (right). (Modified, with permission, from Qian et al. 1998.)
One mode of cell division is asymmetric: The progenitor produces one differentiated daughter and another daughter that retains its stem cell-like properties. This mode does not permit amplification of the stem cell population. In a second mode neural stem cells divide symmetrically to produce two stem cells, and in this way expand the population of proliferative progenitor cells. Both symmetric and asymmetric modes have been found in the embryonic cerebral cortex in vivo and in cortical cells grown in tissue culture (Figure 53-1C).
The incidence of symmetric and asymmetric cell division is influenced by signals in the local environment of the dividing cell, making it possible to control the probability of self-renewal or differentiation. Environmental factors can influence the outcome of progenitor cell divisions in two fundamental ways. They can act in an “instructive” manner, biasing the outcome of the division process and causing the stem cell to adopt one fate at the expense of others. Or they can act in a “selective” manner, permitting the survival and maturation of only certain cell progeny.
Radial Glial Cells Serve As Neural Progenitors and Structural Scaffolds
Radial glial cells are the earliest morphologically distinguishable cell type to appear within the primitive neural epithelium. Their cell bodies are located in the ventricular zone and their long process extends to the pial surface. As the brain thickens, the processes of radial glial cells remain attached to the ventricular and pial surfaces. After the generation of neurons is complete, many radial glial cells differentiate into astrocytes, a prominent class of glial cell in the mature brain. The elongated shape of the radial glial cell places it in a favorable position to serve as a scaffold for the migration of neurons that emerge from the ventricular zone (Figure 53-2).
Figure 53-2 Radial glial cells serve as precursors to neurons in the central nervous system and also provide a scaffold for radial neuronal migration. Progenitor cells in the ventricular zone of the developing cerebral cortex have nuclei that migrate along the apical-basal axis as they progress through the cell cycle. Left: During the G1 phase nuclei rise from the inner (apical) surface of the ventricular zone. During the S phase they reside in the outer (basal) third of the ventricular zone. During the G2 phase they migrate apically, and mitosis occurs when the nuclei reach the ventricular surface. Right: During cell division radial glial cells give rise to postmitotic neurons that migrate away from the ventricular zone using radial glial cells as a guide.
The ventricular zone was once thought to contain two major cell types: radial glial cells and a set of neuroepithelial progenitors, which served as the primary source of neurons. This classical view has changed dramatically in the last few years. Radial glial cells are in fact progenitor cells that generate both neurons and astrocytes in addition to their role in neuronal migration (Figure 53-2). When radial glial cells are selectively labeled with fluorescent dyes or viruses, tracing of their clonal progeny reveals cell clusters that contain both neuronal and radial glial cells. These findings indicate that radial glial cells are able to undergo both asymmetric and self-renewing cell division, and serve as a major source of post-mitotic neurons as well as astrocytes. Radial glial cells may also serve as progenitors of neurons in the adult central nervous system.
The Generation of Neurons or Glial Cells Is Regulated by Delta-Notch Signaling and Basic Helix-Loop-Helix Transcription Factors
How do radial glial cells make the decision to self-renew, generate neurons, or give rise to mature astrocytes? The answer to this question involves an evolutionarily conserved signaling system.
In flies and vertebrates neural fate is regulated by a cell-surface signaling system, comprised of the transmembrane ligand delta and its receptor notch, which regulates a cascade of transcription factors of the basic helix-loop-helix (bHLH) family. This signaling system was revealed in genetic studies in Drosophila. Neurons emerge from within a larger cluster of ectodermal cells, each of which has the potential to generate neurons. This proneural region is defined by expression of proneural genes, which encode transcription factors of the bHLH class. Yet within the proneural region only certain cells form neurons; the others become epidermal support cells.
Initially the ligand delta and its receptor notch are expressed at similar levels by all proneural cells (Figure 53-3A). With time, however, notch activity is enhanced in one cell and suppressed in its neighbor. The cell in which notch activity is highest loses the potential to form a neuron and acquires an alternative fate. The binding of Delta to Notch results in proteolytic cleavage of the Notch cytoplasmic domain, which then enters the nucleus. There it functions as a transcription factor, regulating the activity of bHLH transcription factors that suppress the ability of the cell to become a neuron and reduce the level of expression of the ligand Delta (Figure 53-3B). Through this feedback pathway a minor difference in the initial level of Notch signaling is rapidly amplified to generate an all-or-none difference in the status of Notch activation, and consequently the fates of the two cells. This basic logic of Delta-Notch and bHLH signaling has been conserved in vertebrate and invertebrate neural tissues.
Figure 53-3 Delta acts as a ligand for Notch and determines neuronal fate.
A. At the onset of the interaction between two cells, the ligand Delta engages the receptor Notch. Delta and Notch are expressed at similar levels on each cell, and thus their initial signaling strength is equal.
B. A small imbalance in the strength of Delta-Notch signaling breaks the symmetry of the interaction. In this example the left cell provides a slightly greater Delta signal, thus activating Notch signaling in the right cell to a greater extent. On binding by Delta, the cytoplasmic domain of Notch is cleaved to form a proteolytic fragment called Notch-Intra, which enters the nucleus of the cell and initiates a basic helix-loop-helix (bHLH) transcriptional cascade that regulates the level of delta expression. Notch-Intra forms a transcriptional complex with a bHLH protein, suppressor of hairless, which binds to and activates the gene encoding a second bHLH protein, enhancer of split. Once activated, enhancer of split binds to and represses expression of the gene encoding a third bHLH protein, achaete-scute. Achaete-scute activity promotes expression of delta. Thus, by repressing achaete-scute, enhancer of split decreases transcriptional activation of the Delta gene and production of Delta protein. This diminishes the ability of the cell on the right to activate Notch signaling in the left cell.
C. Once the level of Notch signaling in the left cell has been reduced, suppressor of hairless no longer activates enhancer of split, and the level of expression of achaete-scute increases, resulting in enhanced expression of Delta and further activation of Notch signaling in the right cell. In this way a small initial imbalance in Delta-Notch signaling is rapidly amplified into a marked asymmetry in the level of Notch activation in the two cells. In the mammalian central nervous system cells with high levels of Notch activation are diverted from neuronal fates, whereas cells with low levels of Notch activation become neurons.
How does Notch signaling regulate neuronal and glial production in mammals? At early stages in the development of the mammalian cortex Notch signaling promotes the generation of radial glial cells by activating members of the Hes family of bHLH transcriptional repressors. Two of these proteins, Hes1 and Hes5, appear to maintain radial glial cell character by activating the expression of an ErbB class tyrosine kinase receptor for neuregulin, a secreted signal that promotes radial glial cell identity. The Notch ligand Delta1 as well as neuregulin are expressed by newly generated cortical neurons; thus the radial glial cells depend on feedback signals from their neuronal progeny for continued production.
At later stages of cortical development Notch signaling continues to activate Hes proteins, but a change in the intracellular response pathway results in astrocyte differentiation. At this stage the Hes proteins work by activating a transcription factor, STAT3, which recruits the serine-threonine kinase JAK2, a potent inducer of astrocyte differentiation. STAT3 also activates expression of astrocyte-specific genes such as the glial-fibrillary acidic protein (GFAP).
The generation of oligodendrocytes, the second major class of glial cells in the central nervous system, follows many of the principles that control neuron and astrocyte production. Notch signaling regulates the expression of two bHLH transcription factors, Olig1 and Olig2, which have essential roles in the production of embryonic and postnatal oligodendrocytes.
The generation of cortical neurons requires that cells avoid exposure to notch signals (Figure 53-4). This task is achieved in part through the expression of numb, a cytoplasmic protein that antagonizes notch signaling. The key role of numb in neurogenesis was first shown in Drosophila, where it determines the neuronal fate of daughter cells of asymmetrically dividing progenitors. In the mammalian cortex numb, as with Notch, is preferentially localized in neuronal daughters and antagonizes notch signaling. As a consequence, loss of numb activity causes progenitor cells to proliferate extensively. The inhibition of notch signaling results in the expression of several proneural bHLH transcription factors, notably Mash1, neurogenin-1, and neurogenin-2. Neurogenins promote neuronal production by activating downstream bHLH proteins such as neuroD, and they block the formation of astrocytes by inhibiting JAK and STAT signaling.
Figure 53-4 Notch signaling regulates the fate of cells in the developing cerebral cortex. Notch signaling has several roles in cell differentiation in the developing cerebral cortex. The activation of Notch signaling in glial progenitor cells results in the differentiation as astrocytes and inhibits differentiation as oligodendrocytes (left pathway). Notch signaling also inhibits progenitor cells from differentiating into neurons (right pathway). (Photo on left reproduced, with permission, from David H. Rowitch; middle photo reproduced, with permission, from Edward Nyatia and Dirk Michael Lang/SA Science Lens Competition(SAASTA); photo on right reproduced, with permission, from Masatoshi Takeichi, Riken Center for Developmental Biology.
Although delta-notch signaling and bHLH transcription factor activators lie at the heart of the decision to produce neurons or glial cells, several additional transcriptional pathways augment this core molecular program. One important transcription factor, REST/NRSF, is expressed in neural progenitors and glial cells, where it represses the expression of neuronal genes. REST/NRSF rapidly degrades as neurons differentiate, permitting the expression of neurogenic bHLH factors and other neuronal genes. Homeodomain transcription factors of the SoxB class also play an important role in maintaining neural progenitors by blocking neurogenic bHLH protein activity. The differentiation of neurons therefore requires the avoidance of REST/NRSF and SoxB protein activity.
Neuronal Migration Establishes the Layered Organization of the Cerebral Cortex
The mammalian cerebral cortex develops in three main stages: a preplate, a cortical plate, and finally the mature pattern of layers. Neural precursors in the ventricular zone of the preplate differentiate into neurons that migrate along radial glial fibers before settling in the cortical plate. These migratory cells divide the preplate into a subplate and a marginal zone (Figure 53-5).
Figure 53-5 Neurons migrate along radial glial cells. After their generation from radial glial cells, newly generated neurons in the embryonic cerebral cortex extend a leading process that wraps around the shaft of the radial glial cell, thus using the radial glial cells as scaffolds during their migration from the ventricular zone to the pial surface of cortex.
Once within the cortical plate, neurons become organized into well-defined layers. The laminar settling position of a neuron is correlated precisely with its birthday, a term that refers to the time at which a dividing precursor cell undergoes its final round of cell division and gives rise to a postmitotic neuron. Cells that migrate from the ventricular zone and leave the cell cycle at early stages give rise to neurons that settle in the deepest layers of the cortex. Cells that exit the cell cycle at progressively later stages migrate over longer distances and pass earlier-born neurons, before settling in more superficial layers of the cortex. Thus the layering of neurons in the cerebral cortex follows an inside-first, outside-last rule (Figure 53-6A).
Figure 53-6 The migration of neurons in the cerebral cortex is responsible for the layered organization of the cortex. (Modified, with permission, from Olsen and Walsh 2002.)
A. During normal cortical development neurons use radial glial cells as migratory scaffolds as they enter the cortical plate. As they approach the pial surface, neurons stop migrating and detach from radial glial cells. (CP, cortical plate; MZ, marginal zone; VZ, ventricular zone.)
B. An orderly inside-out pattern of neuronal migration results in the formation of six neuronal layers in the mature cerebral cortex, arranged between the white matter (WM) and subplate (SP).
C. In the mouse mutant reeler, which lacks functional reelin protein, the layering of neurons in the cortical plate is severely disrupted and partially inverted. In addition, the entire cortical plate develops beneath the subplate.
D. In doublecortin (dcx) mutants the cortex is thickened, neurons lose their characteristic layered identity, and some layers contain fewer neurons. A similar disruption is observed in Lis1 mutants, which underlies certain forms of human lissencephaly.
Disruption in the migratory and settling programs of cortical neurons underlies much human cortical pathology (Figure 53–6C, D). In lissencephaly (Greek, smooth brain, referring to the characteristic smoothing of the cortical surface in patients with the disorder) neurons leave the ventricular zone but fail to complete their migration into the cortical plate. As a result, the mature cortex is typically reduced from six to four neuronal layers, and the arrangement of neurons in each remaining layer is disordered. Occasionally, lissencephaly is accompanied by the presence of an additional group of neurons in the subcortical white matter. Patients with lissencephalies from mutations in the Lis1 and doublecortin genes often suffer severe mental retardation and intractable epilepsy. The Lis1 and doublecortin proteins have been localized to microtubules, suggesting they are involved in microtubule-dependent nuclear movement, although their precise functions in neuronal migration remain unclear.
Mutations that disrupt the reelin signaling pathway disrupt the final stage of neuronal migration through the cortical subplate. Reelin is an extracellular matrix protein that is secreted from the Cajal-Retzius cells, a class of neurons found in the preplate and marginal zone. Signals from these cells are crucial for the migration of cortical neurons. In mice lacking functional reelin, neurons fail to detach from their radial glial scaffolds and pile up underneath the cortical plate, disobeying the inside-out migratory rule. As a consequence, the normal layering of cell types is inverted and the marginal zone is lost. Reelin acts through cell-surface receptors that include the ApoE receptor 2 and the very low-density lipoprotein receptor. The binding of reelin to these receptors activates an intracellular protein, Dab1, which transduces reelin signals. Cadherin-like adhesion proteins may also be involved in transducing reelin signals. Not surprisingly, the loss of proteins that transduce reelin signals produce similar migratory phenotypes.
Central Neurons Migrate Along Glial Cells and Axons to Reach Their Final Settling Position
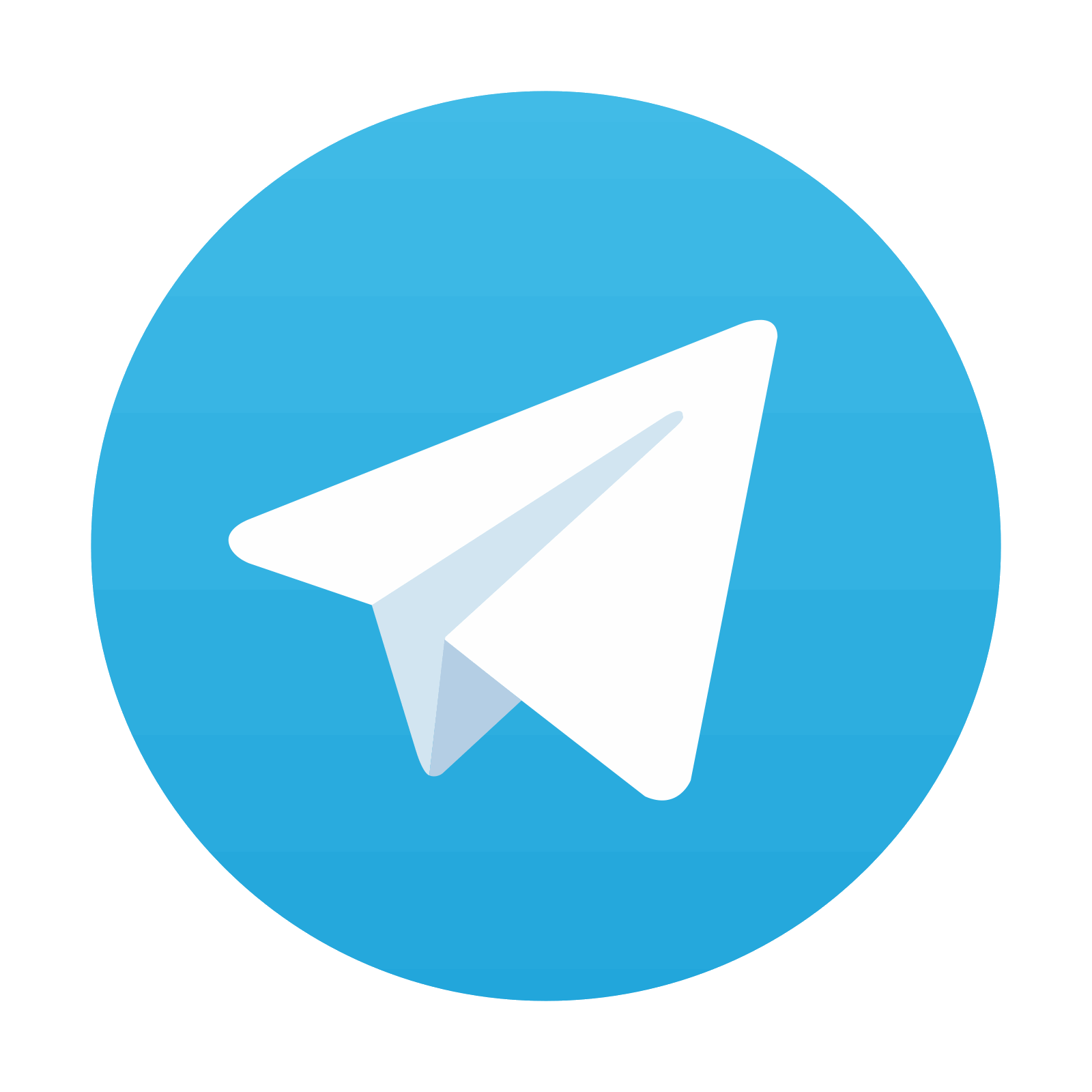
Stay updated, free articles. Join our Telegram channel
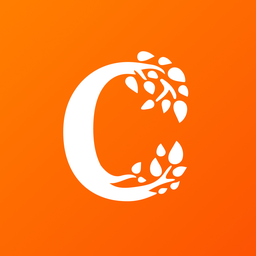
Full access? Get Clinical Tree
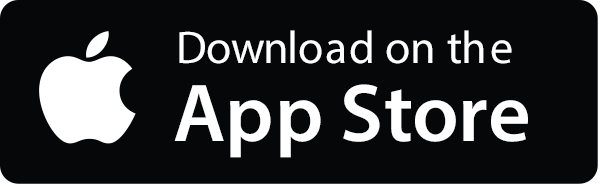
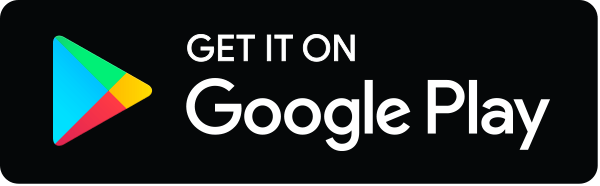