Early Events in the Development of the Cerebral Cortex
Volney L. Sheen
Christopher A. Walsh
Introduction
There is an increasing awareness that many epileptic conditions, particularly in the pediatric age group, are associated with developmental abnormalities of the cerebral cortex. This chapter provides an overview of normal development of the cortex. The developmental description is intended to provide a context for understanding the function of genes which, when disrupted, have been shown to give rise to these malformations of cortical development (MCD). Although the genetic causes and potential mechanisms leading to the MCD are discussed, a more thorough discussion of the pathology of epilepsy is presented in several other chapters.
The cerebral cortex must undergo a series of fundamental developmental processes to create the underlying neuronal scaffolding that is responsible for the cortical organization underlying complex cognitive functions. The development of the enormous diversity of cells of the nervous system spans a series of crucial events, from (a) the division and expansion of neural precursor populations, to (b) the migration of these neurons out of the ventricular zone (VZ) into the cortical plate, and followed by (c) the differentiation of neurons into the various neuronal subpopulations that make up the six-layered cortex.1,40 Disruption of each of these processes has been suggested to give rise to a distinct set of human disorders of cortical development. Disorders of proliferation lead to microcephaly (“small brain”), disorders of initial migration give rise to periventricular heterotopia (ectopic nodules of neurons located along the lateral ventricles), disorders of subsequent migration contribute to classical lissencephaly (“smooth brain”) and subcortical band heterotopia (referring to an additional layer of neurons beneath the cortex), disorders in neuronal arrest giving rise to cobblestone lissencephaly (smooth brain with ectopic nodules of neurons on the surface of the brain), and disorders in neuronal differentiation giving rise to potential abnormal cell connectivity.73 Many of the genes responsible for these disorders have been identified. Moreover, such genes provide an initial foundation with which to understand the normal cellular and molecular mechanisms involved in the development of the cerebral cortex and, ultimately, the mechanisms responsible for some pediatric epilepsies.
Formation of the Mammalian Central Nervous System
Neurogenesis
The oldest neurons in the human cortex appear very soon after the regional definition of the cerebral cortex, at about 33 to 42 days postovulation.54,62 The earliest neurons derive from an epithelium that is originally pseudostratified, in which the neuroepithelial cells have attachments to both the inner, ventricular face and the outer, pial surface. The early neural progenitor cells show a characteristic nuclear movement as the cells progress through the cell cycle: Neurons in the mitotic (M) phase have their nuclei immediately lining the ependymal zone, but as the cells move into synthesis (S)-phase, when they replicate their DNA, their nuclei translocate away from the ventricular lining (growth [G] 1phase). Cells then transition from S to the growth (G) 2phase, at which time their nuclei translocate back toward the ventricular surface and into M-phase. Finally, the nuclei of precursors, which re-enter the cell cycle, move back toward the upper half of the epithelium and away from the ventricular lining during G1 phase (Fig. 1A,B). Although the majority of neurons appear to derive from the ventricular lining, a second proliferative region develops just adjacent to the epithelium, called the subventricular zone. Neurons are generated along the neuroepithelial lining of the ventricular zone and indirectly from intermediate progenitor cells that reside in this region.61
During neural proliferation, precursors undergoing cell division in M-phase either remain as progenitors or adopt a neuronal cell fate. The neural progenitors split into two cells along a cleavage plane that is either vertical (perpendicular to the ependymal lining) or horizontal (parallel to the ependymal lining). Vertical cleavages produce identical daughter cells that resemble the original neural precursors. These symmetric divisions appear to expand or maintain the progenitor pool, because both daughter progenitors re-enter the cell cycle and their nuclei translate away from the lining during G1 phase. In contrast, horizontally dividing cells produce two types of daughter cells—one that behaves like a young migratory neuron and another that remains within the proliferative zone as a neural precursor and re-enters the cell cycle. These asymmetric divisions appear to give rise to the postmitotic neurons that will comprise the overlying cerebral cortex.18
Neuronal Migration
The six-layered adult cortex actually derives from three distinct populations of neurons with very different patterns of development. The oldest cortical neurons form a structure known as the preplate or the primordial plexiform layer.52 When the earliest formed neurons of the preplate complete their final mitosis to become postmitotic, they do not actually migrate from where they are formed; instead, they translocate their nuclei through one cellular process, then retract their processes and begin differentiation into neurons (Fig. 1B).52,54 Later-formed neurons divide the preplate into two layers, called the marginal zone (future layer I) and the subplate (future deep neurons of layer VI and the white matter) (Fig. 1B). These later formed neurons do not migrate directly to the cortex but rather display a retrograde movement toward the ventricle while in the VZ/SVZ, before migration back out into the cortical plate
(Fig. 1B).61 These neurons form the vast majority of all cortical neurons, including cells in layers VI through II, and form a third transient layer called the cortical plate. The cortical plate is first seen at about embryonic day (E) 52 in the human, almost 3 weeks after appearance of the preplate.54 Studies in many different mammals have repeatedly shown that neurons within the cortical plate follow a remarkable and precise sequence of events when inserting into the preexisting preplate.9,67 The oldest neurons of the cortical plate (future layer VI cells) cease migration nearest the subplate cells, whereas the next cohort of cortical neurons (future layer V) migrates just beyond the older layer VI cells to settle nearer the pial surface. Successive waves of cortical plate neurons then all follow the same “inside-out” gradient of neurogenesis (Fig. 1B), with cortical neurogenesis and migration completed by about 24 weeks of embryonic development.54 The cortical-plate neurons are formed at a later time and in the context of a larger brain than are the preplate cells; consequently, they must migrate for distances of up to millimeters—equivalent to hundreds of times their cell body size. This long-distance migration appears to involve mechanisms distinct from those that operate during the formation of the preplate.
(Fig. 1B).61 These neurons form the vast majority of all cortical neurons, including cells in layers VI through II, and form a third transient layer called the cortical plate. The cortical plate is first seen at about embryonic day (E) 52 in the human, almost 3 weeks after appearance of the preplate.54 Studies in many different mammals have repeatedly shown that neurons within the cortical plate follow a remarkable and precise sequence of events when inserting into the preexisting preplate.9,67 The oldest neurons of the cortical plate (future layer VI cells) cease migration nearest the subplate cells, whereas the next cohort of cortical neurons (future layer V) migrates just beyond the older layer VI cells to settle nearer the pial surface. Successive waves of cortical plate neurons then all follow the same “inside-out” gradient of neurogenesis (Fig. 1B), with cortical neurogenesis and migration completed by about 24 weeks of embryonic development.54 The cortical-plate neurons are formed at a later time and in the context of a larger brain than are the preplate cells; consequently, they must migrate for distances of up to millimeters—equivalent to hundreds of times their cell body size. This long-distance migration appears to involve mechanisms distinct from those that operate during the formation of the preplate.
Although the neocortical excitatory neurons originate from the proliferative ventricular zone of the cortex, many of the inhibitory cortical interneurons actually appear to be derived from the germinal zone of the basal ganglia. Most of these cells derive from the medial ganglionic eminence, but may also arise from the caudal and lateral ganglionic eminences, the septal region, and the cortex itself. These interneurons follow a tangential migratory pathway from the ganglionic eminences to the cortex.4,5,84
Table 1 Cortical Malformations and Associated Gene Abnormalities | ||||||||||||||||||||||||||||||||||||||||||||||||||||||||||||||||||||||||||||||
---|---|---|---|---|---|---|---|---|---|---|---|---|---|---|---|---|---|---|---|---|---|---|---|---|---|---|---|---|---|---|---|---|---|---|---|---|---|---|---|---|---|---|---|---|---|---|---|---|---|---|---|---|---|---|---|---|---|---|---|---|---|---|---|---|---|---|---|---|---|---|---|---|---|---|---|---|---|---|
|
Neuronal Differentiation
Although cortical plate neurons are generated and mature gradually, the earlier formed preplate and subplate neurons achieve a high level of morphologic maturity at early cortical stages (E30–E50 in humans).45 In animal models, early subplate cells have been shown to receive the earliest connections from outside the cortex (especially from the thalamus) and to form the earliest efferent connections from the cortex to subcortical sites.3 Remarkably, the high level of morphologic and synaptic maturity achieved by the subplate is transient because these neurons regress, and most appear to die off as a normal morphogenetic process during later cortical development. The transience of the subplate has given rise to the theory that subplate cells are “pioneer” neurons that are present to guide and direct later-formed definitive cortical connections. Thus, the regression and death of subplate connections may be a necessary, perhaps even obligatory, step in normal cortical development.3
Within the cerebral cortex, the progressive differentiation of a given neuron can be divided into sequential phases. Some of the steps in neuronal development include the initial neuronal specification while in the ventricular zone and several subsequent phases of postmigratory neuronal differentiation, including formation of an axon and dendrites, synthesis of synaptic machinery, and expression of ion channels. Simple invertebrate organisms with small nervous systems show specific genetic mutations that affect each sequential step in neuronal development, suggesting that the steps occur in a defined sequence.21 Although, for any given neuron, these developmental steps occur in series; for the cortex as a whole, the developmental steps do not represent entirely separate steps because cortical neurons are also formed in sequence. Thus, the elaboration of neurites in the oldest cortical neurons overlaps in time with the specification of newer cells, and so on.
Despite the overlap in the formation of cortical neurons of different layers, cell biologic study of vertebrate cortical neurons suggests that each neuron undergoes a fairly predefined sequence of developmental steps. For example, neuronal precursors appear to receive information about the specific type of neuron they will become during their final cell division and before they migrate out of the proliferative region of the cortex. Previous transplantation studies have suggested that, once a postmitotic neuron has obtained a neuronal fate and commitment to form one particular cell type, it will carry out its developmental program and migrate to an appropriate cortical location, even if it is challenged by having to migrate through a novel cellular environment.53 A number of transcription factors that are activated in a cortical progenitor will determine its cortical neuron phenotype and promote the repression of certain genetic sequences that would specify a different cell fate.27 Once the neurons have obtained their laminar position, cortical pyramidal cells and interneurons make and receive efferent and afferent projections. These processes depend on the interplay between intrinsic transcriptional factors and extrinsic signaling, thereby establishing the appropriate topographic intercortical and intracortical connections and proper connectivity with the thalamus.66
Malformations of Cortical Development
The complexity of proliferation, migration, and differentiation in the developing cortex make it hardly surprising that disruptions in these processes result in a number of neuropathologic disorders, including epilepsies (Table 1). Although malformations of the cortex are discussed in detail in Chapters 14 and 259, we present an overview of some genetically inherited
disorders to illustrate how our improved understanding of the basic biology of cortical development improves our insight into epileptic pathology.
disorders to illustrate how our improved understanding of the basic biology of cortical development improves our insight into epileptic pathology.
Disruption of Neurogenesis
When the capacity to generate the numbers of cells needed by the developing cerebral cortex is impaired, not surprisingly, the human brain becomes microcephalic. Many genetic and nongenetic factors can result in a small brain. For example, congenital infection with toxoplasma, maternal alcohol overconsumption, as well as general disorders of cell metabolism or cholesterol synthesis can lead to a small brain. In general, however, disruption of such processes are readily apparent on examination and also affect various organ systems, leading to extra-central nervous system (CNS) manifestations.58,63 For this reason, disorders that cause microcephaly vera (true microcephaly) have been used to more clearly delineate processes that directly affect cortical development. In such cases, the brain size is greater than two standard deviations below the norm (Fig. 2A vs. 2B). The disorder is associated with mental retardation, there is gross preservation of the brain structures (except on a smaller scale), and the disease occurs in the absence of findings outside the CNS.57,58 Identification of the genes that cause macrocephaly vera indicates that some of them have direct relevance to neurogenesis.
Genes causal for microcephaly (Microcephalin, ASPM, CDK5RAP2, and CENPJ) have been suggested to play some role in neurogenesis along the ventricular neuroepithe- lium,12,13,83 and appear to play direct roles in the mechanisms underlying cell division. Microcephalin is a gene that contains three BRCA1 domains that have been implicated in cell-cycle control and DNA repair.39 Loss of DNA repair genes can lead
to increased programmed cell death, thereby suggesting that loss of Microcephalin function leads to increased cell loss along the ventricular neuroepithelium. More recent studies have suggested two potential roles for Microcephalin protein: One in the control of cell-cycle timing and the other in the control of DNA repair (following ionizing radiation damage).78,85 ASPM (abnormal spindle-like, microcephaly-associated) is a gene that encodes a very large protein. In Drosophila, ASPM is essential for normal mitotic spindle function in embryonic neuro- blasts by stabilizing the tubulin ring complexes that organize the centrosomes.22 CDK5RAP2 and CENPJ are also centrosomal proteins, localized to the centrosomes in interphase and to the spindle poles during mitosis.13,38
to increased programmed cell death, thereby suggesting that loss of Microcephalin function leads to increased cell loss along the ventricular neuroepithelium. More recent studies have suggested two potential roles for Microcephalin protein: One in the control of cell-cycle timing and the other in the control of DNA repair (following ionizing radiation damage).78,85 ASPM (abnormal spindle-like, microcephaly-associated) is a gene that encodes a very large protein. In Drosophila, ASPM is essential for normal mitotic spindle function in embryonic neuro- blasts by stabilizing the tubulin ring complexes that organize the centrosomes.22 CDK5RAP2 and CENPJ are also centrosomal proteins, localized to the centrosomes in interphase and to the spindle poles during mitosis.13,38
Although mental retardation has been a uniform feature seen in microcephaly vera,83 seizures have not been included in the primary clinical phenotype, despite the high prevalence of epilepsy in microcephaly patients. More recent findings, however, have shown that mutations in ASPM can lead to epilepsy in some affected individuals. Seizures have onset at 2 to 3 years of age, and they appear focal in onset. EEGs demonstrate a generalized slowing interspersed with occasional Rolandic or central spike activity.75 In other patients with macrocephaly, severe disruption of the gyral pattern and neuronal architecture occurs, and these conditions (known as macrocephaly with simplified gyri) are generally associated with early-onset seizures and early death; genes for these syndromes have not been identified yet.
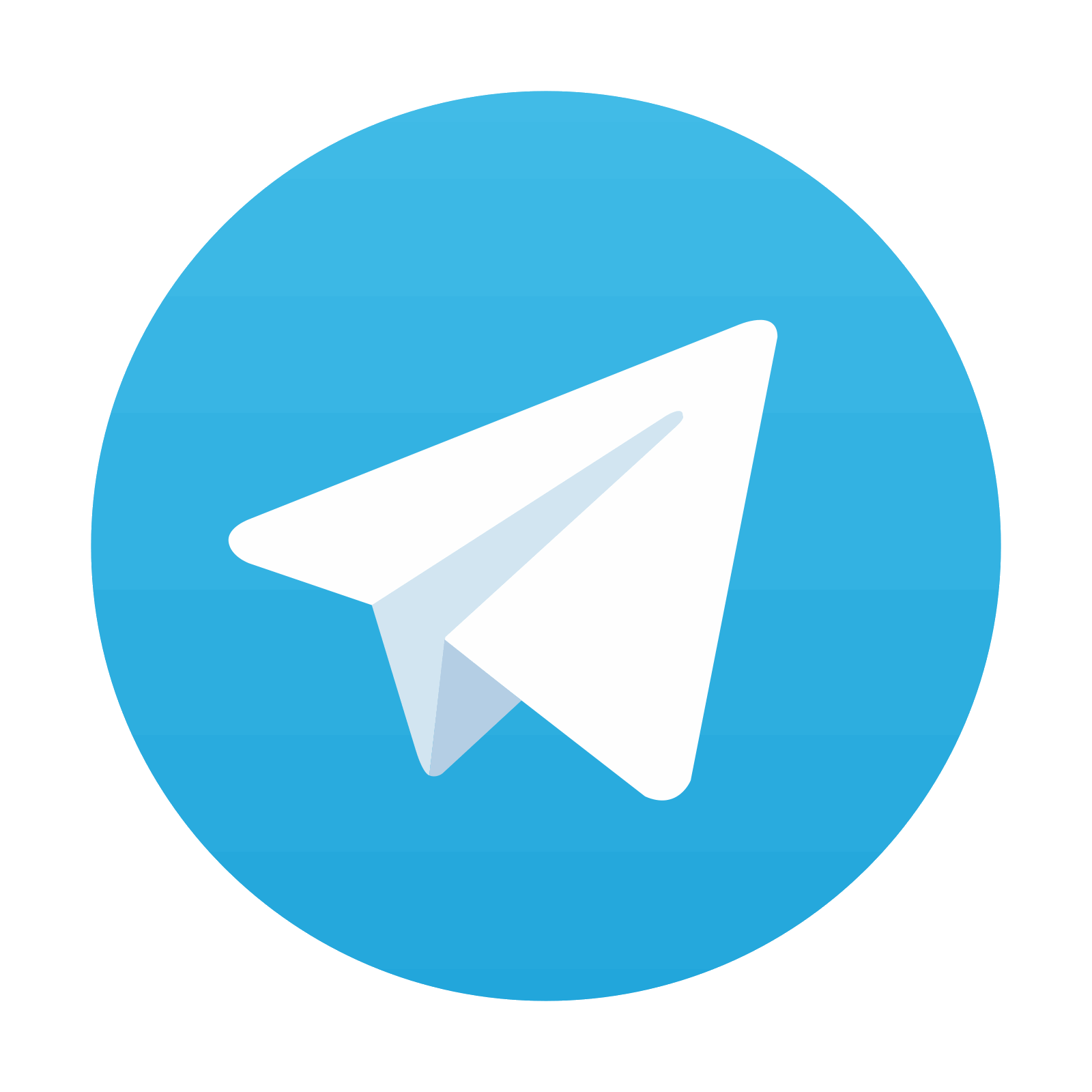
Stay updated, free articles. Join our Telegram channel
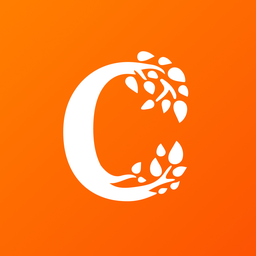
Full access? Get Clinical Tree
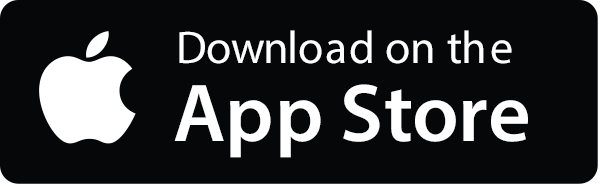
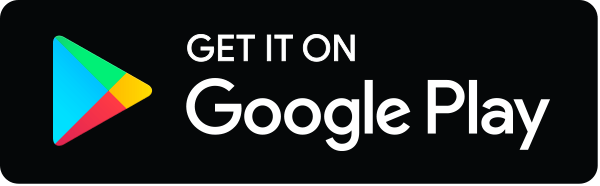