Fig. 18.1
Scalp EEG voltage recordings resulting from an excitatory input on a deep synapse. EPSP excitatory postsynaptic potential. Modified from Kandel and Schwartz [69]
Methods of EEG Recording
Amplification of the exceedingly minute electracerebral voltage changes into interpretable waveforms is the function of the EEG equipment (Fig. 18.2). EEG voltage changes are transmitted by the electrodes and conducting gel applied to the scalp through electrode wires, which connect the electrodes to the jack box of the EEG equipment. While different types of electrodes are available, most standard laboratories employ gold cup electrodes with holes in the center and silver–silver chloride electrodes for EEG recordings. Silver–silver chloride electrodes need repeated chloriding for proper maintenance. Positive and negative charges are generated between the scalp and recording electrode as a result of ionic dissociation in the electrodes. Paste or conducting gel is used to secure the electrodes. The electrode–electrolyte interface is the most critical link in the EEG machine, as most artifacts originate at this site; careful preparation is therefore very important. The impedance in a pair of electrodes should be measured by an impedance meter and should be less than 5000 (5 K) ohms. High impedance impairs the ability of the electrical signal to reach the amplifier and interferes with the capacity of the amplifier to eliminate environmental noise, thus increasing artifacts [7]. Impedance and impedance-related artifacts are described in greater detail in Chap. 17.


Fig. 18.2
Computer-generated components of a digital polygraph
Electrode Placement, Channels, and Montages
The placement of the electrodes is determined by the 10–20 electrode placement system which is recommended by the International Federation of Societies for EEG and Clinical Neurophysiology and was published by Jasper in 1958 [8]. This system is based on definable anatomical landmarks (see Fig. 24.1) and consists of letters denoting the parts of the brain underneath the area of the scalp and numbers denoting specific locations. The odd numbers refer to the left side of the head, and the even numbers refer to the right side. Important landmarks for measuring include the inion, the nasion, and the right and left preauricular points. The distance from the nasion to inion along the midline through the vertex should be measured. FPz in the midline is 10 % above the nasion of the total distance between the inion and nasion. The electrodes marked FP1 and FP2 are located laterally 10 % above the nasion of the total distance between the inion and nasion measured along the temporal regions through the preauricular points. Oz denotes an electrode placed at a distance of 10 % above the inion of the total distance between the nasion and inion. T3 and T4 electrodes are placed in a location 10 % above the preauricular points of a total distance between the two preauricular points. The rest of the electrodes are located at a distance of 20 % measured from inion to nasion anteroposteriorly or laterally through the ears as well as transversely between the ears. The nomenclature was recently changed to rename T3, T4, T5, and T6 to T7, T8, P7, and P8, respectively, in what has been designated the “Modified Combinatorial Nomenclature.”
Two electrodes connected to each other constitute a channel or a derivation (e.g., F7-T3 is a channel). A montage refers to the manner in which these channels are arranged. Both bipolar montages (connection of the electrodes between two relatively active sites over the scalp; e.g., F7-T3) and referential montages (connection of the electrodes between an active and relatively inactive site; e.g., F7-M1, F8-M2, and T4-Cz) are recommended. Electrode inputs for digital systems always include one or more additional inputs for reference electrodes. A location between Fpz and Fz is often chosen for the reference electrode but any location on the scalp that is relatively electrically neutral and where firm attachment to the scalp is possible can be used. Reference electrodes are necessary because digital EEG recording is always referential.
Despite the pivotal role that EEG plays in the analysis of sleep, the limited number of inputs available on most PSG equipment results in a less than desirable number of channels dedicated to EEG. In fact, the American Academy of Sleep Medicine (AASM) guidelines [9] call for a minimum of just three channels that record from a single hemisphere and which do not record from the temporal lobes; such a limited montage would result in an inability to capture focal epileptiform activity arising from the most common location for such discharges (e.g., temporal lobes), as well as missing focal abnormalities from the hemisphere not being recorded [10]. Given the pro-epileptogenic effect of drowsiness and nonrapid eye movement (NREM) sleep, and the fact that nocturnal seizures that are often mistaken for parasomnias and are sometimes encountered during an in-laboratory PSG, it would be unfortunate if a clinically important finding were to be missed or improperly interpreted because of an inadequate number of EEG channels.
For this reason, in our own laboratory, we use between 4 and 8 EEG channels including temporal leads from both hemispheres (Table 18.1); such montages increase the yield of capturing focal or diffuse slow-waves or epileptiform activities compared to recording with 2–4 channels. When nocturnal seizures are suspected, an extended EEG montage (Table 18.2; Fig. 18.3), covering bilateral temporal and parasagittal regions and including both bipolar and referential channels is recommended. Full complement of electrodes and special electrode placements (e.g., Tl and T2 electrodes) should be used. Simultaneous video recording (video-PSG study) for correlation of EEG activities with the actual behavior of the patients is crucial. In computerized PSG recordings (digital PSG recordings), which are currently performed in most laboratories, it is easy to change the recording speed from the standard 10 mm per second of the usual sleep recording to 30 mm/s of the standard EEG recording for proper identification of epileptiform discharges.

Table 18.1
Typical overnight polysomnographic montage used in our laboratory
Channel number | Name |
---|---|
1 | F3-M2 |
2 | C3-M2 |
3 | T3-M2 |
4 | O1-M2 |
5 | F4-M1 |
6 | C4-M1 |
7 | T4-M1 |
8 | O2-M1 |
9 | Left electro-occulogram (E1-M2) |
10 | Right electro-oculogram (E2-M2) |
11 | Chin electromyogram (EMG) |
12 | ECG |
13 | Heart Rate |
14 | Left gastrocnemius EMG |
15 | Left tibialis anterior EMG |
16 | Right gastrocnemius EMG |
17 | Right tibialis anterior EMG |
18 | Intercostal EMG |
19 | Oronasal thermistora |
20 | Nasal pressure transducera |
21 | Chest |
22 | Abdomen |
23 | Snoring |
24 | Arterial oxygen saturation |
Table 18.2
Extended EEG (“seizure”) montage
Channel number | Name |
---|---|
1 | F4-M1 |
2 | C4-M1 |
3 | O2-M1 |
4 | C3-M2 |
5 | Fp1-F7 |
6 | F7-T3 |
7 | T3-T5 |
8 | T5-O1 |
9 | Fp2-F8 |
10 | F8-T4 |
11 | T4-T6 |
12 | T6-O2 |
13 | Fp1-F3 |
14 | F3-C3 |
15 | C3-P3 |
16 | P3-O1 |
17 | Fp2-F4 |
18 | F4-C4 |
19 | C4-P4 |
20 | P4-O2 |
21 | Left electro-oculogram (E1-M2) |
22 | Right electro-oculogram (E2-M2) |
23 | Chin electromyogram (EMG) |
24 | Right Masseter EMG |
25 | Left biceps EMG |
26 | Right biceps EMG |
27 | Left tibialis anterior EMG |
28 | Right tibialis anterior EMG |
29 | Intercostal EMG |
30 | Oronasal thermistora |
31 | Nasal pressure transducera |
32 | Chest |
33 | Abdomen |
34 | Snoring |
35 | Arterial oxygen saturation |
36 | ECG |
37 | Heart Rate |

Fig. 18.3
a A 10-s and b 30-s epoch from the polysomnogram of a 32-year-old man referred to the sleep laboratory for possible OSA. He has a history of epilepsy; hence, an extended EEG (“seizure”) montage was requested. Normal awake EEG pattern characterized by symmetrical, sinusoidal posterior dominant alpha rhythm at 9–10 Hz is noted. The top four channels are referential channels connected to the left mastoid. The next 16 are bipolar channels arranged in a double banana montage covering parasagittal and temporal regions as per the International 10–20 electrode placement system. E1-M1 and E2-M1, electrooculogram (EOG) channels; Masseter1-Masseter2 and Chin1-Chin2, masseter (right) and submental electromyogram (EMG); ECG, electrcardiogram; HR, heart rate; Int1-Int2, intercostal EMG; LtArm1-LtArm2; RtArm1-RtArm2, left and right biceps EMG, LTIB; RTIB, left and right tibilais anterior EMG. OroNs1-OroNS2, oronasal airflow (thermistor); PFlow, nasal pressure transducer; Chest and ABD, effort belts; SaO2, arterial oxygen saturation by finger oximetry. Also included is a snore channel. ECG artifact is noted in the referential leads and EOG leads. Reproduced with permission from: Chokroverty and Thomas [65]
Differential Amplification and Sensitivity
An understanding of the physiological basis of recorded waveforms is important. While a detailed description of the elementary concepts of electricity, resistance, and capacitance is provided in Chap. 16, and an overview of digital equipment and recording techniques with regards to PSG is provided in Chap. 17, a review of a few key concepts is provided here, especially with regards to EEG recording and analysis. EEG equipment measures and amplifies potential differences, which are always measured between two points; in this case, it is the potential difference between the two scalp electrodes. This is referred to as differential amplification. This is an extremely important concept to understand. Figure 18.4 provides an illustration. If electrode A (the “active” electrode) is connected to input 1 of an amplifier and electrode B (the “reference” electrode) is connected to input 2 of the same amplifier, the amplifier would determine the difference between the two inputs (in this case, 5 µV) and there would be a deflection of 5 units. The actual amount of the deflection in millimeters would depend on the sensitivity (see below). Similarly, the direction of the deflection (upwards or downwards) depends on the relative polarity of the two electrodes (as discussed below). The key concept here is that the differential amplifier is displaying the difference between the two electrode inputs rather than absolute voltages; if the two electrodes are at the same voltage, there would be no deflection at all. This does not mean that neither site is electrically active, just that there is no difference in the voltages between the two. This is a useful feature, because environmental noise, which is likely to be the same at the two electrodes, is “subtracted out” and therefore does not contaminate the recording. The common mode rejection ratio measures the ability of the amplifier to suppress a signal, such as noise, that is present simultaneously at both electrodes (Fig. 18.5). This ratio should exceed 1000 to 1; most amplifiers currently in service have values that exceed 10,000 to 1. On the other hand, electrodes that are too close to each other may miss significant electrocerebral activity because the area involved is large enough to involve both almost equally; thus the need to reference to a distant electrically inactive site or to use double-distance electrodes as is done, for example, in brain death studies. Analyzing the same EEG using different reformattable montages helps increase the sensitivity of the study overall.



Fig. 18.4
Illustration of the concept of differential amplification. Electrode A, at 10 µV, is connected into input 1 of the differential amplifier, and Electrode B, at 15 µV, is connected into input 2, resulting in the creation of channel A-B. A potential difference of 5 µV results in a corresponding deflection of 5 units in channel A-B. In this case, the deflection is negative or upwards; the direction in any given situation would depend on the polarity convention (see Figs. 18.6 and 18.7 for details)

Fig. 18.5
A schematic representation of common mode rejection amplification. The potential difference between the electrodes connected to input 1 and input 2 results in a single amplified upwards deflection. The complex underlying waveform is common to both electrodes, is artifactual and not of biological interest, and represents “noise” that is canceled out by the common mode rejection amplifier. Reproduced and modified with permission from Libenson [5]
Amplifiers can faithfully amplify input voltages only within a certain range known as the dynamic range. Input voltages below the lower limit of the dynamic range are lost in noise; voltages above the upper limit result in a distorted EEG output. Flexible control of amplification within the dynamic range is achieved by manipulating the sensitivity switch. The sensitivity switch is connected to a series of voltage dividers that attenuate the amplified cerebral voltages sufficiently for the EEG record to be interpretable. Sensitivity is defined as the amount of voltage necessary to produce a prespecified output deflection. The usual units are microvolts per millimeter or millivolts per centimeter. In the past, studies were mostly recorded with analog systems, where sensitivity had to be adjusted by the technician as the study was being recorded and could not be manipulated by the polysomnographer at the time of interpretation. With virtual replacement of analog with digital systems, the polysomnographer has the freedom to change sensitivity as best suited to the situation. Chapters 16 and 17 discuss analog and digital systems in greater detail.
Polarity Convention
Whereas the sensitivity settings determine the amplitude of waveforms, the positivity or negativity of cerebral activity determines their polarity. The differential amplifier compares relative positivity and negativity at the two electrodes. The resulting deflection is determined according to the polarity convention. If input 1 is negative relative to input 2, or if input 2 is positive relative to input 1, there is an upward, or negative deflection. On the other hand, if input 1 is positive relative to input 2, or if input 2 is negative relative to input 1, there is a downward, or positive deflection. When a number of inputs are connected together as channels in a bipolar EEG montage, the direction of the deflections produced according to the above polarity convention results in the deflections “meeting” at a common point, referred to as a phase reversal, which can be used to roughly localize the scalp distribution of those waveforms. Such a phase reversal is due to an area of cortical negativity, although the direction of deflection in any given channel depends on whether the first electrode in the channel is closer or further away from this area of cortical negativity in comparison to the second, resulting in a negative or positive deflection, respectively (Fig. 18.4).
An illustrative example of this concept is provided in Fig. 18.6, which represents a bipolar hypothetical left parasagittal chain. If one were to assume that there is an area of relative cortical negativity at C3 (as typically occurs in epileptogenic foci), then there would be a downward deflection in F3-C3 (because C3 is more negative than F3) and an upward deflection in C3-P3 (because P3 is less negative, or more positive, than C3). However, in practice, epileptogenic foci are rarely so precisely localized, and the area of cortical negativity generally extends beyond a single electrode, i.e., it usually has a “field”. Thus, while C3 may be the most negative electrode, its adjacent electrodes, while less negative than C3 itself, are nevertheless more negative than the electrodes farther away from C3 in the montage. The result is that F3 is more negative than Fp1 (causing a positive or downward deflection in Fp1-F3), and P3 is more negative than O1 (causing a negative or upward deflection in P3-O1). Reviewing the entire montage, it is obvious that while a large portion of the left frontotemporal region is involved, the phase reversal is at C3, the area of maximum cortical negativity. The “meeting point” is between the F3-C3 and C3-P3 electrodes, and the common electrode between the two, the site of phase reversal, is C3.


Fig. 18.6
Schematic representation of bipolar montage with an area of cortical negativity at the C3 electrode. There is a phase reversal at C3, the area of maximal cortical negativity. Note that while the maximum deflection is noted in the channels containing the phase reversal (F3-C3 and C3-P3), adjacent channels (FP1-F3 and P3-O1) also show defections predicted by the relative negativity of the individual electrodes, thereby producing a “field.” Reproduced and modified with permission from Libenson [5]
In a referential montage, where each electrode is connected to a common relatively electrically inactive reference electrode (e.g., right mastoid, M2), the same epileptogenic focus would look different. Since every electrode recording from the area of cortical negativity would be more negative than the reference electrode, deflection would always be upwards in channels where M2 is connected to input 2 as is the convention. However, since the degree of deflection of the waveform is dependent on the potential differences between the two electrodes, the amplitude would be highest in that channel where the active electrode is the most negative. Thus, in the example above, the amplitude of the negative potential is highest at C3, the same electrode where a phase reversal occurred on the bipolar montage. But because the area of cortical negativity extends beyond C3 to involve the adjacent electrodes, although to a lesser degree, deflections occur in those channels as well, i.e., there is a field noted even on the referential montage (Fig. 18.7). Thus, while the channel containing the most negative electrode (C3-M2) demonstrates the negative wave with the highest amplitude, adjacent electrodes (F3-M2 and P3-M2) demonstrate negative waves with lower amplitudes, thus producing a field. The ability to view a suspicious waveform (e.g., a spike or sharp wave of potential epileptic significance) in multiple reformattable montages is a benefit of digital recordings.


Fig. 18.7
Schematic representation of a referential montage with an area of cortical negativity at the C3 electrode. The montage has been created by connecting electrodes Fp1, C3, P3, and O1 to the contralateral mastoid (M2, not shown). All channels demonstrate negative (upward) deflections, with the waveform being of highest amplitude in the channel containing the electrode with the highest degree of cortical negativity (C3-M2). Adjacent channels demonstrate negative deflections of lower amplitudes, thereby producing a “field.” Reproduced and modified with permission from Libenson [5]
To summarize, localization of an area of epileptogenic potential or an area showing a focal slow wave, as represented by cortical negativity, is by visualization of phase reversal on bipolar montages, and by maximum waveform amplitude on referential montages.
Filters
Once inputs are subtracted and amplified, the result is passed through a series of filters. The goal of filtering is to attenuate voltages occurring at undesirable frequencies (e.g., environmental noise) without disturbing frequencies found in the biological signal of interest. Filters are described in greater detail in Chap. 16. Briefly, however, a high-pass filter, (also known as a low-frequency filter), allows higher frequency activity to pass unchanged while progressively attenuating lower frequencies (see Fig. 16.12a). This is particularly useful to eliminate artifacts of low frequency such as sweat and respiratory artifact. A low-pass filter (also known as a high-frequency filter), allows lower frequencies to pass unchanged while progressively attenuating higher frequencies (see Fig. 16.12b). This is particularly useful to eliminate high-frequency artifacts such as muscle artifact. A 60-Hz or notch filter is also present in most polygraphic amplifiers. This filter is designed to specifically attenuate the 60-Hz artifact from electrical lines very harshly while attenuating activity of surrounding frequencies less extensively. A similar 50-Hz notch is available in European and certain other countries to match the current in those countries. The notch filter should be used sparingly for at least two reasons. Some biological signals of interest to the polysomnographer have waveforms with important components in the range of 40–80 Hz. Examples include myogenic activity and epileptiform spikes, both of which may be significantly attenuated by the notch filter. For example, use of the notch filter in the chin EMG channel may result in a false impression that tonic EMG has significantly decreased. In addition, the capability of the differential amplifier to reject common signals (see above) should be sufficient to suppress 60-Hz artifact in most cases. Thus, the appearance of 60-Hz artifact usually signals a problem somewhere in the polygraphic circuit that needs to be resolved. Most often the culprit is high impedance at the electrode–scalp interface. Less frequently, defects in the amplifier or grounding of the polygraph are responsible. In these cases, addressing the cause of the 60 Hz rather than using the notch filter is the appropriate course. There are circumstances in which a nearby source of 60 Hz (e.g., a critical piece of medical equipment that cannot be disconnected) renders the EEG uninterpretable. Use of the 60-Hz filter may be justified in these circumstances but must be clearly documented.
Analog and Digital Systems
Until digital PSG recording became the norm, analog systems were used. The analog PSG and EEG machines consisted of an oscillograph in the form of a galvanometer pen unit, with current flow from the amplifier entering a coil attached to the unit, inducing a magnetic field that interacted with the permanent magnetic field in which the coil was placed and causing the deflection of the pen. These have been virtually phased out at this time in favor of digital equipment and hence will not be discussed further.
Modern digital communications technology has allowed miniaturization of what had been much bulkier (if somewhat hardier) solid state components. It has also resulted in some reorganization of the functions of the polygraphic circuit. Digital polygraphs perform all of the functions of the analog polygraphic circuit. However, the ability to digitize the EEG signals allows easier manipulation, transmission, display, and storage that confers some distinct advantages. The ability of the polysomnographer to change sensitivities, montages, and filters while reviewing the study, rather than be limited by decisions taken by the technician at the time the study was recorded, is probably the biggest such benefit.
Recorded signals are still analog signals and need to be presented to the analog to digital converter (ADC). The ADC assesses the voltage of the continuous analog EEG, creates a numerical value corresponding to this voltage, and stores this value in memory. The ADC then repeats this process at a uniform interval (intersample interval). In this manner, a continuous (analog) EEG signal is converted into a series of numerical values representing the voltage of the signal at serial moments in time. Thus, the signal is commonly referred to as “digitized” or a “digital signal.” As described in Chap. 16, the reliability of the digital signal is dependent on the sampling rate; if the digitized signal is to reflect the analog signal faithfully, the sampling rate must be at least twice the highest frequency in the analog signal to prevent aliasing (see Figs. 16.17 and 16.18).
The digitized signal is now passed to a computer for storage in memory and further manipulation by a software program. Software programs perform many of the functions of the solid state components of the analog polygraph.
Filtering is an important function performed by software on the digitized EEG signal. Digital filters are computer algorithms that transform digitized EEG by filtering out designated frequencies. Digital filtering [11] can be performed in the frequency domain by computing the Fourier transform of a segment of EEG, replacing coefficients at the frequency one wishes to eliminate by zero, and then reconstituting the EEG by computing the inverse Fourier transform (see further discussion of Fourier analysis below). Digital filtering can also be performed in the time domain by using a moving average method. Such finite impulse response filters are increasingly used in digital EEG machines and allow filtering without phase distortion, an advantage over traditional analog filters [12].
Software can manipulate and analyze digitized EEG signal in more sophisticated ways as well. Spectral analysis is a commonly employed technique relying on the Fourier theorem and forms the basis of sleep stage scoring software. Pitfalls and limitations of this technique are discussed below. Algorithms designed to detect seizures and epileptiform abnormalities are also commercially available [13]. The clinical utility of these is variable and at present they do not replace a thorough analysis of the original record by a qualified interpreter.
Modified and organized digital signal can be directed from computer memory to a variety of destinations. Polygraphic data must be presented to the interpreter for visual inspection. Polygraphic data in digital systems is typically displayed on monitors. Monitor resolution must be sufficient so that the degree of resolution provided by the ADC is not significantly compromised. For example, if 1024 pixels (a common horizontal resolution in “off the shelf” computer monitors) is available to display 30 s of EEG, at most 1024/30 or 34 pixels can be devoted to display one second of EEG. This is far less than the 256 samples per second horizontal resolution provided by ADCs typically used in contemporary polygraphic systems. While this degree of horizontal resolution may be adequate for sleep stage scoring, it is not sufficient for analysis of epileptiform activity or electrographic seizures. Changing the timebase of the display so that 10 s epochs are displayed on the monitor will triple the horizontal resolution of the monitor in the above example and bring it more into line with the resolution provided by the ADC. Similar considerations pertain to the vertical resolution of the monitor though this is of less concern in polygraphy where less channels of polygraphic recording are typically presented at any time. In general, larger monitors with higher monitor resolution better reflect all the information present in the digitized signal.
Digitized polygraphic data can be transmitted to a printer to generate a “hard copy” polygraphic tracing of selected epochs. Printing an entire polygraph is rarely necessary with digital systems. Digitized data in computer memory must ultimately be transmitted to peripheral devices for storage. A variety of digital storage mediums are available, all of which are less expensive and more convenient than the paper and microfilm required for analog EEG. After security and privacy issues are addressed, digitized polygraphic data can be transmitted via network or the internet to other computers in the clinic, the interpreter’s home, or on another continent.
Advantages and Limitations of Digital Recording Systems
Digital recording systems are now the norm and have rendered analog polygraph systems obsolete. With digital systems, there has been a revolution in techniques of data acquisition, display, and storage. Some of these have been alluded to in the discussion above, but to summarize, digital equipment is much less bulkier and conserves space. Previously recorded data can be manipulated retroactively and changes can be applied to the filter settings, sensitivities, and monitor speeds. As a result, artifacts can often be minimized and eliminated, data can be analyzed in multiple ways, and areas of interest can be more easily pinpointed and logged for future reference. Computerized recording makes the EEG paperless, conserving space, and being more environmentally friendly. Digital information is easier to store on inexpensive media such as DVDs, and the digital format translates more easily into databases. For these reasons, digital recording will undoubtedly continue to replace analog polygraphic systems over time.
Spectral Analysis
Spectral analysis is probably the most widely used computerized analysis of digitized EEG [14]. Spectral analysis is based on the Fourier theorem, which states that any waveform can be decomposed into a sum of sine waves at different frequencies with different amplitudes and different phase relationships. When summed, these waves reconstitute the original waveform. The Fourier transformation is a mathematical operation that provides the frequency, amplitude, and phase parameters of each of these component sine waves. Fourier coefficients represent the amplitude and phase relationship at each of the component sine-wave frequencies. Squaring and summing the Fourier coefficients at each frequency provides the power at that frequency. A plot of power at each of the component frequencies is called the power spectrum. The power spectrum allows determination of relative amounts of given frequencies in the waveform over the time segment analyzed.
The fast Fourier transform algorithm allows real-time spectral analysis with contemporary personal computers. Commercially available software packages offer straightforward presentation of the power contained in the traditional frequency bands during a set period of EEG. This allows detection and quantification of frequencies not detected with visual inspection. However, there are many potential pitfalls. Theoretically, the power spectrum is a faithful representation of the original signal only if the original signal is stationary (has stable statistical properties). The EEG signal is clearly not stationary over long periods, although it appears reasonably stationary over brief epochs. In practice, this means that the EEG segment selected for analysis should not include obvious changes such as those due to alerting or drowsiness. In addition, the Fourier theorem assumes that an infinitely long sample is available for analysis. Because, even long samples are clinically impractical, tapering, or “windowing” of the endpoints of the sample is necessary to attenuate the spurious frequencies (leakage) arising from the segmentation of the signal. Windowing is never completely successful—some leakage is unavoidable. This may affect clinical interpretation when power is displayed in the traditional frequency bands; for example, a reasonable amount of alpha power may leak to the theta band or beta bands. Nonsinusoidal rhythms, such as “spiky alpha” are common in routine EEG. Fourier analysis of a nonsinusoidal rhythm of a set frequency often shows a large peak at that frequency with smaller peaks at harmonics of the frequency. These smaller, higher frequency peaks may lead the interpreter to conclude that cerebral activity at the higher frequency is actually present. The most common pitfall in interpreting power spectra is artifact. Artifact is ubiquitous, often subtle, and can take an almost infinite variety of forms. The computer cannot separate artifact from EEG and includes artifact in the computation of the power spectrum. This can lead to significant misinterpretation. Artifact is much more difficult to recognize in the power spectrum than in the unprocessed EEG. It is therefore very important to review EEG before spectral analysis or interpretation of the power spectrum to prevent analysis of segments contaminated by artifact [15, 16].
Despite these limitations, spectral analysis can play a useful role in the operating room and in routine scoring of sleep studies. A basic understanding of the principles of signal processing and thorough experience in the appearance of various cerebral activities after spectral analysis is necessary. Unprocessed “real” physiologic signal must always be reviewed. Spectral analysis has not demonstrated any consistent clinical utility in routine EEG despite almost two decades of active research. Because the potential for misinterpretation and abuse is high, the major neurologic and neurophysiologic professional organizations have taken strong positions against the use of spectral analysis during routine EEG.
Electro-oculography
Electro-oculography (EOG) recording is crucial to staging sleep accurately. Gold cup or silver–silver chloride electrodes can be used to monitor the EOG. The sensitivity and filter settings for EOG are similar to those used for EEG (see Table 18.3). The two recommended electrodes are labeled E1 (1 cm below the left outer canthus) and E2 (placed 1 cm above the right outer canthus) both referenced to the right mastoid [9]; this arrangement allows simultaneous recording of both vertical eye movements (such as blinking) and horizontal eye movements (both slow and rapid) (Fig. 18.8).

Table 18.3
Filter and sensitivity settings for polysomnographic studies
Characteristics | High–frequency filter (Hz) | Time-constant (s) | Low–Frequency filter (Hz) | Sensitivity |
---|---|---|---|---|
Electroencephalogram | 70 or 35 | 0.4 | 0.3 | 5–7 μV/mm |
Electro-oculogram | 70 or 35 | 0.4 | 0.3 | 5–7 μV/mm |
Electromyogram | 90 | 0.04 | 5.0 | 2–3 μV/mm |
Electrocardiogram | 15 | 0.12 | 1.0 | 1 mV/cm to start; adjust |
Airflow and effort | 15 | 1 | 0.1 | 5–7 μV/mm; adjust |

Fig. 18.8
a The voltage field generated by the eye can be represented by a simple dipole, the cornea being positive and the retina negative. b With the use of two polygraphic channels to detect conjugate eye movements according to the scheme suggested in the sleep scoring manual, eye movements result in out-of-phase potentials in the two channels
The underlying concept is that the eye is an electric dipole, with relative positivity at the cornea and a relative negativity at the retina. Any eye movement changes the orientation of the dipole, and it is the movement of the dipole that is recorded as a potential difference between the two electrodes used to record the EOG. In this arrangement, conjugate eye movements produce out-of-phase deflections in the two EOG channels whereas the EEG slow activities contaminating the eye electrodes are in phase. For example, when the eyes look to the right (see Fig. 18.8), the cornea of the right eye approaches electrode A in input 1 and electrode A becomes positive relative to the inactive ear. According to the polarity convention, amplifier 1 will register a downward deflection. Simultaneously, the retina of the left eye approaches electrode B connected to input 2. Consequently, electrode B becomes negative relative to the inactive ear and amplifier 2 registers an upward deflection. The out-of-phase deflections in the two adjacent channels indicate that a conjugate eye movement has occurred. Similarly, an upward eye movement results in a downward deflection in amplifier 1 and an upward deflection in amplifier 2. Eye blinks will produce an identical pattern because eye closure results in an upward rotation of the eyeball (Bell’s phenomenon).
Eye movements are general characteristic of the sleep stage in which they occur and are an essential part of scoring. Eye blinks, seen only in wakefulness, are conjugate vertical eye movements occurring at 0.5–2 Hz with the eyes open or closed. REMs (conjugate, irregular, sharp eye movements with an initial deflection of less than half a second) occur in wakefulness along with high chin EMG tone, eye blinks and a posterior dominant rhythm, but also occur in REM sleep, especially in phasic REM where they occur in bursts seen in all directions (horizontal, oblique, and vertical) and are accompanied by low to absent chin EMG tone (interspersed with similar phasic bursting) and a desynchronized, amorphous EEG pattern. The frequency with which bursts of REMs occur in REM sleep, measured as REMs/minute, is called REM density. It typically increases in later REM cycles during the course of a normal PSG; this may be reversed in patients with depression. Slow lateral eye movements (SEMs or SLEMs) are seen in drowsiness and light sleep and are defined as conjugate, sinusoidal, and regular eye movements with an initial deflection of greater than half a second (Fig. 24.9). These eye movements are not under voluntary control and cannot be volitionally simulated. In patients, who do not generate a posterior dominant rhythm, their appearance heralds Stage N1 sleep. While they may persist into Stage N2 during the early part of the night, they generally disappear in Stage N3 and REM sleep. However, patients on antidepressants such as selective serotonin reuptake inhibitors (SSRIs such as fluoxetine and paroxetine) as well as serotonin–norepinephrine reuptake inhibitors (SNRI such as duloxetine) may have unusual eye movements that appear to be a mixture of rapid and slow eye movements occurring well into Stage N3 and often into REM sleep (colloquially referred to among polysomnographers as “Prozac eyes”); their presence makes sleep staging difficult (Fig. 18.9) and can render scoring of a multiple sleep latency testing (MSLT) equally frustrating. The role of EOG in sleep staging is further discussed in Chap. 24.


Fig. 18.9
A 60 s epoch from the overnight polysomnogram of a 35-year-old man complaining of excessive daytime sleepiness and with a prior diagnosis of obstructive sleep apnea. He also has a history of depression and is on citalopram, a selective serotonin reuptake inhibitor (SSRI). This epoch represents Stage N2 sleep, as evidenced by the presence of K complexes and sleep spindles. Note the presence of excessive eye movements, representing a combination of slow and rapid eye movements. Eye movements generally do not persist into Stage N2 and beyond but are often seen in patients on SSRIs (colloquially referred to as “Prozac eyes”). Top eight channels; EEG recording with electrodes placed according to the 10–20 international electrode placement system. Chin1-Chin2, submental electromyogram (EMG); ECG, electrcardiogram; HR, heart rate; LTIB; RTIB, left and right tibilais anterior EMG; LGAST; RGAST, left and right gastrocnemius EMG; OroNs1-OroNS2, oronasal airflow; Pflw1-Pflw2, nasal pressure transducer recording; Chest and ABD, effort belts; SaO2, arterial oxygen saturation by finger oximetry. Also included is a snore channel. Reproduced with permission from: Chokroverty and Thomas [65]
Santamaria and Chiappa [17] found two types of eye movements in drowsiness, not previously reported, by placing recording electrodes over the globe in addition to traditional EOG; they described small fast irregular eye movements in 60 % of normal subjects in early drowsiness, preceding slow eye movements, as well as small fast rhythmic eye movements in 30 % of normal subjects, usually associated with the traditional slow eye movements. The former did not appear in traditional EOG recordings, and when the latter occasionally did, they were of very low amplitude. These have yet to be validated by other authors.
Electromyographic Recordings in Sleep Disorders
Electromyography (EMG) channels provide important physiological characteristics that help determine sleep stage, as well as aiding in the diagnosis and classification of a variety of parasomnias [18]. At a minimum, a PSG consists of chin EMG channels recording activity from the mentalis and submental muscles (the mylohyoid and anterior belly of the digastric, supplied by the motor fibers of the trigeminal nerve), and bilateral leg EMG channels recording activity from the tibialis anterior muscles. EMG is recorded using a gold cup or a silver–silver chloride electrode applied to a clean surface using tape or electrode glue. For chin EMG recordings, at least three EMG electrodes are applied so that in the event of a problem with one of the electrodes the additional backup electrode can be connected during the recording without disturbing the patient. The electrode impedance should be less than 5 K. The high- and low-frequency filter settings for the EMG recordings are different from those used for EEG and EOG and are listed in Table 18.3. The sensitivity should be at least 20 μV/cm for mental or submental EMG activity.
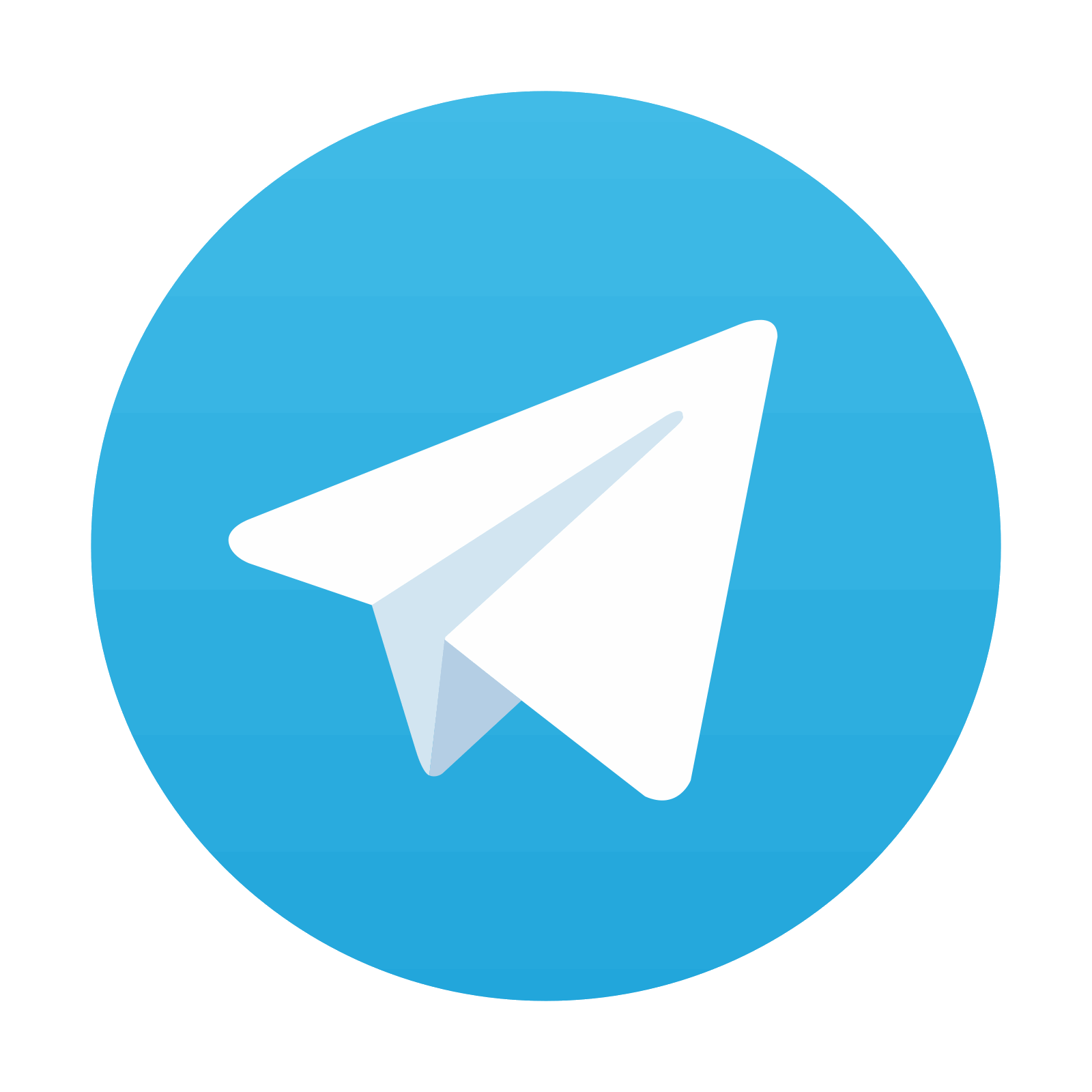
Stay updated, free articles. Join our Telegram channel
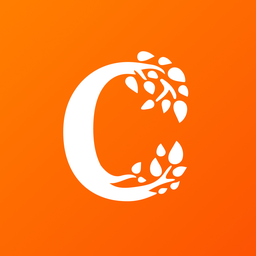
Full access? Get Clinical Tree
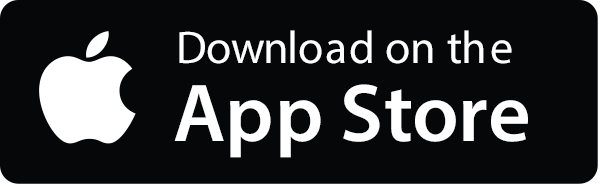
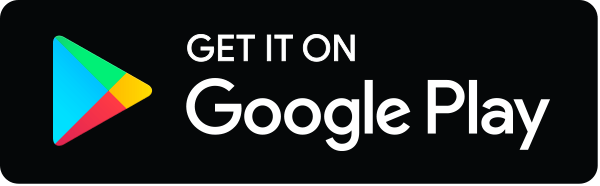