Fig. 18.1
Transport of water, gases, and electrolytes across the blood–brain barrier. Small lipophilic molecules passively diffuse through endothelial cells, while the transport of electrolytes and water is tightly regulated by transmembrane carriers
Water readily crosses the BBB by diffusion, at least in part through aquaporin-4 channels, and moves freely in or out of the brain according to the osmotic gradient, whereas sodium and chloride ions depend on the combined action of an array of transporters (Fig. 18.1), with an exchange half-time of sodium ions of approximately 2 h. Thus, acute variations of plasma osmolality might cause a substantial shift of water across the BBB. When plasma osmolality decreases abruptly, as in acute hyponatremia, the movement of water driven by the osmotic gradient from the vascular compartment to the brain causes an expansion of the cerebral interstitial fluid. This leads to a decrease in extracellular tonicity and secondary swelling of neurons and astrocytes. The opposite phenomenon, shrinkage of interstitial and cellular volumes, occurs with an abrupt increase in plasma osmolality. Progressive changes in plasma osmolality do not have the same consequences as cells are able to regulate their volume and osmolality. This regulation is based on the detection of volume changes and the activation of mechanisms of transport of electrolytes and organic osmoles, a process that can take 24–72 h. When a new equilibrium is reached, as in chronic hypernatremia, a rapid correction of plasma osmolality may thus be detrimental.
The BBB is impermeable to calcium, phosphorus, and magnesium ions . Their cerebrospinal fluid (CSF) levels (Table 18.1) are mostly determined by the secretion of a fluid of constant composition by the choroid plexus. CSF levels of calcium are approximately half of the plasma concentration and there is only a weak correlation between plasma and CSF levels. Magnesium is found in greater concentrations in the CSF than in the plasma and there is no clear correlation between CSF and plasma levels. Phosphorus levels in the CSF are approximately 60% of plasma levels. It should be noted that the immaturity of the blood–brain barrier in neonates allows calcium and magnesium ions to cross and their CSF levels correlate with their plasma levels [6].
Table 18.1
Electrolyte and acid-base composition of the CSF
CSF | CSF/plasma ratio | Exchange half-time | |
---|---|---|---|
Sodium | 141.2 ± 6.0 (mmol/l) | 0.95 | 1 h |
Potassium | 2.96 ± 0.45 (mmol/l) | 0.70 | 24 h |
Chloride | 120.3 ± 3.3 (mmol/l) | 1.10 | |
Osmolality | 287.2 ± 5.5 (mOsm) | 1.00 | |
Magnesium | 2.71 ± 0.11 (mg/dL) | 1.40 | |
Calcium | 4.56 ± 0.32 (mg/dL) | 0.50 | |
Phosphorus | 0.60 | ||
pH | 7.32 ± 0.09 | 7.40 ± 0.06 | |
pCO2 | 44 (mmHg) | 40 | Seconds |
![]() | 22 (mmol/l) | 24 | Hours to days |
Water | 12–25 s |
The BBB is relatively impermeable to protons and bicarbonate ions, but it is very permeable to CO2 gas molecules. CO2 is also produced by neural cell metabolism. The pCO2 is thus usually a few mm Hg higher and the pH slightly lower in the CSF than in the arterial blood. Changes in arterial pCO2, by altering pH, activate homeostatic mechanisms that return the pH back toward normal. These mechanisms involve the adjustment of bicarbonate levels in the CSF and progressively take place over a period of several hours, whereas changes in CSF pCO2 occur within minutes. Thus, compensation for changes in the pH of the CSF requires hours. Also, in a patient with an acute metabolic acidosis (decrease in plasma bicarbonate concentration) and a compensatory respiratory alkalosis (decrease in arterial pCO2), the brain will be paradoxically alkalotic.
Neuronal Excitability : Membrane Potential, Ion Channels, Neurotransmitters, and Their Receptors
A seizure is the manifestation of the abnormal synchronous activation of a group of neurons. This is thought to be caused by an imbalance between neuronal excitation and inhibition. Recruitment of neurons during seizure can occur not only by excitatory glutamate-dependent synaptic transmission but also by extra-synaptic ephaptic coupling, a process during which local extracellular electrical fields generated by adjacent neurons feedback onto the electrical potential across the neuronal membrane. Inhibitory gamma-hydroxy-butyric acid (GABA)-dependent synaptic transmission represses seizure initiation and propagation (Fig. 18.2). Both synaptic and extra-synaptic transmission can be disrupted by changes in the electrolyte and acid-base composition of the extracellular fluid.


Fig. 18.2
Role of electrolytes in synaptic transmission. Sodium and chloride ions are the main ions involved in the generation of excitatory post-synaptic potentials (EPSP) and inhibitory post-synaptic potentials (IPSP), respectively. Calcium ions participate in the fusion of pre-synaptic vesicles and in neurotransmitter (both glutamate and GABA) release and contribute to the generation of EPSP, while magnesium ions limit the opening of glutamate NMDA channels
Extracellular low osmolality and hyponatremia increase neuronal excitability through various mechanisms [7–10]. To some extent, they enhance excitatory neurotransmission at the pre- and post-synaptic levels by facilitating calcium uptake [11, 12]. But the most important effect of low osmolality, whether it is caused or not by hyponatremia, occurs through cellular swelling, which enhances ephaptic coupling [7, 10, 13]. Additional effects of astrocytic swelling include the disruption of glutamate-glutamine metabolism and the release of excitatory amino acids by astrocytes [14, 15]. Low extracellular chloride concentrations also contribute to increase excitability by depressing GABA-dependent inhibitory currents [16]. Hyperosmolality has the reverse effect and decreases neuronal excitability [17].
Calcium and magnesium ions both are voltage membrane stabilizers. They screen surface negative charges on neuronal membranes and regulate the activation of voltage-gated ion channels [9, 18]. There is an inverse correlation between extracellular calcium concentrations and neuronal excitability [19]. Immersing hippocampal slices in a low-calcium solution provokes the occurrence of spontaneous synchronized bursts similar to epileptiform discharges [20]. This is at least in part explained by an increase in resting membrane potential and a decrease of the firing threshold [21] and occurs even when excitatory neurotransmission is blocked [22]. Conversely, a high-calcium solution completely prevents seizure-like activity in hippocampal slices [23]. Similar findings are observed when manipulating extracellular magnesium concentrations in vitro. Sporadic epileptiform bursts followed by ictal discharges develop when hippocampal slices are bathed in a low-magnesium solution [23–26]. Calcium and magnesium ions act as antagonists at all chemical synapses. Calcium ions enter the pre-synaptic axon terminals through voltage-gated channels and bind to protein complexes, allowing the fusion of vesicles to the synaptic membrane and the release of neurotransmitters into the synaptic cleft (Fig. 18.2) [27–29]. Calcium entry through ligand-gated channels at the post-synaptic levels, such as the glutamate N-methyl-d-aspartate (NMDA) receptors, also contributes to excitatory neurotransmission. On the other hand, magnesium ions limit the influx of calcium ions through pre-synaptic voltage-dependent channels and thus modulate neurotransmitter release [25]. In addition, they also regulate synaptic transmission at the post-synaptic level by gating glutamate NMDA receptors in a voltage-dependent manner. At normal resting membrane potential, magnesium ions occupy a site inside the channel part of the receptor and prevent the influx of sodium and calcium ions. If synaptic excitation through other types of glutamate receptors (alpha-amino-3-hydroxy-5-methyl-4-isoxazolepropionic acid [AMPA] and kainate) causes sufficient depolarization, magnesium ions translocate out of the channels, which open [26, 30, 31].
While both synaptic potentiation and membrane destabilization are necessary to generate epileptiform discharges and seizures in the low-magnesium model of seizures [23], synaptic transmission is not necessary and in fact abolished in the low-calcium condition [32]. Neuronal synchronization thus has to occur in an ephaptic manner or through gap junctions.
The effect of pH and protons on neuronal excitability has received relatively little attention compared to other ions [33]. Current evidence indicates that the binding of protons to membrane proteins affects their conformation and their functional properties. Proteins whose activity is modulated by pH include voltage-gated ion channels, GABA receptors, NMDA receptors, gap junctions, calcium-sensing, and pH cation channels [16–24]. Acidosis has little influence on resting membrane potential [34] or axonal conduction [35] but decreases synaptic transmission by both pre- and post-synaptic mechanisms [34–36] and can suppress seizures [37, 38].
Water-Sodium Imbalance and Seizures
Epidemiology and Causes of Sodium and Osmotic Imbalance in Critically Ill Patients
Dysnatremia and water imbalance are frequent in critically ill patients. In a recent study on a large prospective cohort, dysnatremia was observed in 29% of patients [39]. Severe hyponatremia and hypernatremia are, however, much more rare, occurring in 1.2%, and 0.6% of patients, respectively, in another recent large survey [40].
Mechanisms of Sodium and Water Homeostasis
Body water content and sodium concentration are tightly regulated despite variable dietary intake, metabolic activity, and environmental factors. Total body water (TBW) is about 60% of body weight, two thirds of which are intracellular. Osmolarity is defined by the amount of solutes per liter of body fluid and is usually similar to osmolality, which is the amount of solutes per kg of fluid. Plasma osmolarity is mostly determined by sodium levels:
![$$ Osm=2\left[ serum\kern0.5em N{a}^{+}\right]+\frac{\left[ Glucose\right]}{18}+\frac{\left[ BUN\right]}{2.8} $$](https://i0.wp.com/neupsykey.com/wp-content/uploads/2017/08/A145499_3_En_18_Chapter_Equa.gif?w=960)
![$$ Osm=2\left[ serum\kern0.5em N{a}^{+}\right]+\frac{\left[ Glucose\right]}{18}+\frac{\left[ BUN\right]}{2.8} $$](https://i0.wp.com/neupsykey.com/wp-content/uploads/2017/08/A145499_3_En_18_Chapter_Equa.gif?w=960)
Fluid and sodium balance is preserved by the kidneys. Daily fluid intake is about 2–2.5 L, which is needed to replace losses from urine and insensible losses (sudation and perspiration). Daily urine output averages 1.5 L but adults with normal kidney function may excrete as little as the 200 mL of concentrated urines that are necessary to excrete the nitrogenous waste of cellular metabolism, and as much as 25 L in case of excessive fluid intake. Insensible losses represent 500–800 mL/day in a 70-kg adult, but they can substantially increase with fever (50–75 mL/day for each degree celsius of temperature elevation above normal), excessive muscular activity (exercise, convulsions), and heat exposure. Gastrointestinal losses are usually negligible, except when marked vomiting or diarrhea occurs.
Water excretion in the kidneys is regulated by vasopressin (antidiuretic hormone or ADH), which increases the expression of aquaporin channels and water reabsorption in the collecting ducts of the kidney. Vasopressin is produced by neurons in the supraoptic and paraventricular nuclei in the hypothalamus that project to the posterior pituitary gland. Its release is stimulated by increased plasma osmolality (through receptors in the hypothalamus), reduced blood volume (through baroreceptors in the carotid sinuses, veins and atria), angiotensin II (see below), and stress.
Water intake is regulated by thirst, which is triggered by receptors located in the anterolateral hypothalamus in response to increased plasma osmolality or decreased body fluid volume. Water ingestion decreases plasma osmolality, which in turn inhibits vasopressin secretion, allowing the kidneys to dilute urine . With dehydration, plasma osmolality rises, yielding the opposite effect. The system can also be activated by a loss of blood volume or a drop in blood pressure (such as in hemorrhage or extravasation).
The renin-angiotensin system provides an additional mechanism of water-sodium homeostasis. A drop in blood volume and pressure will decrease kidney perfusion, stimulating the release of renin by the juxtaglomerular cells. Renin cleaves angiotensinogen, an inactive pro-peptide, into angiotensin I, a minimally active peptide, which is then converted to angiotensin II by the angiotensin-converting enzyme (ACE) in lung capillaries. Angiotensin II acts by constricting afferent and efferent glomerular arterioles, effectively lowering glomerular blood flow and urine output while preserving glomerular filtration rate and facilitating water reabsorption. It also directly stimulates the reabsorption of sodium along the proximal tubule and loop of Henle. Finally, angiotensin II also causes the release of vasopressin by the pituitary and of aldosterone by the adrenal cortex. Aldosterone stimulates the reabsorption of sodium, in exchange for potassium, and of water from the urine by the distal tubules and collecting ducts. This increases blood volume and, therefore, blood pressure.
Angiotensin II and aldosterone thus act together to stimulate sodium and water reabsorption by the kidney and increase blood pressure. Their effects are opposed by natriuretic peptides (including the atrial natriuretic peptide [ANP] and the brain natriuretic peptide [BNP]), which are released by cardiomyocytes in response to high blood volume and excessive stretching [41, 42].
Hyponatremia
Hyponatremia is defined as plasma sodium concentration <135 mM/L. It may occur with low, normal, or high plasma tonicity (see Fig. 18.3).


Fig. 18.3
Diagnostic algorithm of hyponatremia
Hyponatremia is the most common electrolyte disturbance and affects 12–38% patients admitted to a general ICU [39, 43, 44] and up to 50% of neurological and neurosurgical patients [45, 46]. Age, trauma, coma, and severity of illness are known risk factors. It is associated with increased in-hospital mortality, both if present on admission to the ICU [40] or if acquired during the ICU stay [47, 48], and particularly if corrected too fast or too slow. Even mild dysnatremia increases the risk of in-hospital mortality [40, 49]. Hyponatremia is also associated with longer ICU stay and a longer duration of mechanical ventilation [48, 50].
The most common causes of hyponatremia in critically ill patients are inappropriate fluid replacement and the syndrome of inappropriate antidiuretic hormone (SIADH) . It is often the result of pneumonia [50] or of an acute brain injury, which itself can trigger seizures. In critically ill neurological patients, SIADH and the cerebral salt wasting syndrome (CSWS) are the two most common causes of hyponatremia [51]. Those that have been associated with acute seizures include SIADH, excessive fluid loading, extensive burns, vomiting and drugs [52], bowel preparation with polyethylene glycol, and bladder preparation for endoscopy [53]. There also rare reports of seizures due to water intoxication and exercise-induced hyponatremia [54], intoxication with 3,4-méthylènedioxy-méthamphétamine [55], and psychogenic polydipsia, which can be aggravated by the use of antipsychotic drugs [56].
CSWS has never clearly been identified as a cause of seizures and the underlying cerebral disorder is usually incriminated [57]. Some authors have also suggested that status epilepticus may be involved in the etiology of cerebral salt wasting, as natriuretic peptides levels increase during convulsions [58].
SIADH is the consequence of a direct lesion or dysfunction of the hypothalamus-pituitary axis or of the unregulated secretion of ADH or an ADH-related peptide by a malignancy. Frequent causes are listed in Table 18.2. The increase in ADH release results in excess water reabsorption and expansion of the extracellular fluid volume with hypotonic hyponatremia. Thus SIADH is a volume-expanded state although most patients do not show clinical signs of hypervolemia [51]. ANP is released due to vasopressin stimulation and due to overfilling of the plasma volume [59, 60].
Table 18.2
Causes of SIADH
CNS disorders | • Infections/inflammation (meningitis, encephalitis, AIDS, malaria) • Hemorrhage (SAH, subdural hematoma) • Traumatic brain injury • Guillain–Barre syndrome • Ischemic stroke • Shy–Drager syndrome |
Pulmonary disorders | • Infections (bacterial viral, tuberculosis, aspergillosis) • Cystic fibrosis • Asthma |
Malignancy | • CNS tumors (neuroblastoma) • Carcinoma (lung, gastrointestinal, genitourinary tract) • Lymphoma • Sarcoma (Ewing’s sarcoma) |
Medications stimulating the release of ADH | • SSRIs • Tricyclic antidepressants • Carbamazepine, oxcarbazepine • Phenytoin • Valproic acid • Lamotrigine • Cytotoxins (vincristine, cyclophosphamide, methotrexate, platinum compounds) • NSAIDs • Antipsychotics (phenothiazides, butyrophenones) • Opiates • MDMA • Interferon |
Medications with ADH effects | • Desmopressin • Vasopressin • Oxytocin • Prostaglandins |
Other causes | • Hereditary (mutation of V2 receptor) • Chronic inflammation • Prolonged exercise • Nausea • Pain, stress • Idiopathic • General anesthesia |
Key features for the diagnosis of SIADH include low serum osmolality of <275 mOsm/kg, high urinary osmolality of >100 mOsm/kg, and persistent urine sodium excretion >20 meq/L. SIADH is associated with low uric acid levels (<4 mg/dL) and low blood urea nitrogen (BUN) concentrations (<5 mg/dL) (Table 18.3).
Table 18.3
Comparison of SIADH and CSWS
SIADH | CSW | |
---|---|---|
Fluid balance | Positive | Negative |
Sodium balance | Neutral or positive | Negative |
Extracellular fluid volume | ↑ | ↓ |
Clinical signs of dehydration | Absent | Present |
Central venous pressure | ↔ or ↑ | ↓ |
Weight | ↔ or ↑ | ↓ |
Urine volume | ↔ or ↓ | ↔ or ↑ |
Blood analysis • Sodium • Osmolality • Albumin • Bicarbonate • BUN • Hematocrit • Uric acid | <135 mM/L <285 mOsm/L ↔ ↔ or ↓ ↔ or ↓ ↔ ↔ or ↓ | < 135 mM/L <285 mOsm/L ↑ ↑ ↑ ↑ ↔ or ↓ |
Urine analysis • Sodium • Osmolality | ↑ >200 | ↑↑ >200 |
SIADH should be differentiated from the situation of non-osmotic appropriate release of ADH stimuli, as can be encountered with hypovolemia, hypotension, positive-pressure ventilation, pain, and stress.
Another important of differential diagnosis to consider in patients who present with seizures and SIADH is limbic encephalitis, especially the type associated with antibodies to voltage-gated potassium channel complex [61].
CSWS is a hyponatremic and volume depleted state secondary to a renal loss of sodium and a decrease in extracellular fluid volume due to an intracranial disorder [62]. The mechanism by which cerebral disease results in renal sodium loss is still unclear. Two possible mechanisms have been suggested: impaired sympathetic neural input to the proximal tubule and direct release of natriuretic peptides. The resulting hypovolemia stimulates secondary ADH secretion. CSWS is typically encountered in neurosurgical patients or patients with intracranial disease or injury, especially SAH and meningitis [42, 63].
Hyponatremia is especially frequent after aneurysmal subarachnoid hemorrhage, occurring in approximately 30–56% cases, and is associated with a higher risk of delayed cerebral ischemia [64], but its etiology remains a matter of controversy [65]. SIADH is probably the main cause but CSWS, inadequate fluid replacement, and acute cortisol deficiency have also been implicated [65, 66].
The clinical expression of hyponatremia is related to the severity and acuteness of onset. Symptoms usually become manifest when serum concentrations fall suddenly (<48 h; rate of decrease > 0.5 meq/L/h) below 125 meq/L, as rapid changes of tonicity can exceed the capacity of the brain to adapt its solute content and regulate its volume (see above) [67]. Symptoms of hyponatremia include headache, nausea, vomiting, muscle cramps, and gait disturbance. Confusion and lethargy may ensue. With severe hyponatremia (≤120 meq/L), serious complications from cerebral edema can occur, including coma and eventually death from brain herniation [68].
As previously mentioned, hyponatremia is one of the most common causes of new-onset seizures in hospitalized and critically ill patients [3–5].
The risk of seizures increases with decreasing sodium levels. In a single-center retrospective study of hospitalized patients with hyponatremia, the absolute risk of seizures was 0.7, 2.5, 5.3, and 10.8% for serum sodium values of 120–124, 115–119, 110–114, and <110 meq/L, respectively [52]. Another study found a 7% risk of seizure in patients with chronic hyponatremia and serum sodium level ≤110 meq/L but a 30% risk in patients with acute hyponatremia of this severity, highlighting the fact that not only the absolute value but also the rate at which sodium levels decrease is important [40, 69].
One should remember that mild hyponatremia in patients with chronic epilepsy might not be the cause of seizure but rather the simple consequence of anti-seizure treatment with drugs that cause SIADH, such as oxcarbazepine, carbamazepine, or valproic acid. However, this drug-induced hyponatremia can occasionally be severe enough to exacerbate epilepsy and precipitate seizures [70].
Hyponatremia-induced seizures are most commonly of the generalized tonic-clonic type [4, 52] but focal motor seizures, myoclonic seizures, complex partial seizures, and SE and absence SE have also been reported [4, 52, 71–73].
Seizures seem to be particularly common in children, with studies reporting a 27–66% seizure rate among children with hyponatremia [74, 75]. The majority of seizures in children present as SE. Water intoxication, associated with excessive sweating or gastroenteritis, is the most frequent cause [76, 77]. Severe respiratory syncytial virus infection may also be associated with hyponatremia and seizures [78]. Hyponatremia due to SIADH or CSW is an independent risk factor for post-neurosurgical seizures in children [79].
Management of Hyponatremia
The medical management of hyponatremia can be challenging.
The appropriate management of hyponatremia begins with a complete workup to determine the etiology (Fig. 18.3). Traditional algorithms rely on the clinical assessment of volume status, which can be difficult, and recent studies indicate that they allow physicians to reach a correct diagnosis in only a minority of cases [80]. Recent guidelines advocate the use of an alternative algorithm emphasizing urine electrolytes analysis [81]. It should be noted though that there are no gold standard cut-off values for urinary sodium and urine osmolality [46, 51, 60].
Another limitation of urine electrolytes analysis is that it cannot reliably differentiate SIADH and CSWS because both are characterized by elevated urine osmolality and sodium concentration. They are often difficult to distinguish as they have many features in common (Table 18.3). However, this distinction is important to make as their management strongly differs. It is mostly the presence of signs of volume depletion (hypotension, elevated hematocrit, and BUN/creatinine ratio) despite inappropriately high urine sodium concentration that suggests CSWS. When available, central venous pressure (CVP) (<5 mmHg) and pulmonary capillary wedge pressure (<8 mmHg) are good indicators of circulating blood volume. A negative sodium balance estimated over a period of one or several days supports the diagnosis of CSWS [68, 82]. When in doubt, the response to isotonic saline may also help distinguish between both entities. Restoration of euvolemia in CSWS should remove the stimulus to ADH release, resulting in a dilute urine and correction of the hyponatremia.
In contrast, isotonic saline often worsens the hyponatremia in SIADH as the salt is excreted and some of the water is retained.
Finally, it is important to remember that SIADH and CSWS sometimes succeed each other or occur simultaneously in the same patient and lack of urinary dilution upon isotonic saline administration does not necessarily exclude CSWS.
The correction method depends of the severity of hyponatremia. Treatment is indicated in the presence of acute symptomatic hyponatremia. The risks of hypotonicity should be balanced against those of therapy, as the correction of chronic hyponatremia can lead to neurological injury. Detailed recommendations on the treatment of hyponatremia have been recently published [81].
Severe symptomatic hyponatremia associated with alteration of consciousness or seizures should be quickly but cautiously managed with hypertonic saline solution, even before an etiological diagnosis is made. Intravenous infusion of 150 mL of NaCl 3% through a central venous line should be administered over 20 min in order to achieve an increase of 5 mM/L in serum sodium concentration. This can be repeated if needed. If symptoms improve, the patient can then be managed with isotonic saline until improvement or serum sodium concentration increases by 10 mM/L or reaches 130 mM/L. If symptoms do not improve, management is pursued with 3% hypertonic saline until improvement or serum sodium concentration increases by 10 mM/L reaches 130 mM/L. In both cases, the increase in serum sodium concentration should not exceed 10 mM/L during the first 24 h and 8 mM/L during the subsequent 24 h in order to avoid the risk of osmotic demyelination syndrome, which itself can cause seizures [83–85]. Close monitoring (every 4–6 h) of serum sodium levels is warranted. If over-rapid correction occurs, reversal using desmopressin and water is recommended [86].
Hyponatremia with mild or moderate symptoms can be managed less aggressively and should be guided by the cause. In the presence of moderate symptoms (nausea, headache, confusion) or if hyponatremia developed acutely, immediate administration of 3% saline is suggested.
Once symptoms improve or serum sodium concentration reaches 130 mM/L, management should focus on determining and treating the cause of the hyponatremia (Fig. 18.3). In addition, general measures such as avoiding hypotonic fluids, treating aggravating factors such as pain, nausea, and avoiding medications that can further decrease serum sodium concentration should be taken.
Hyponatremia with volume depletion should be treated by titration of isotonic saline solution with limited parallel free water intake.
Patients with CSWS need to receive isotonic or hypertonic saline to restore their intravascular volume, as long as sodium wasting persists. Colloids can be used when they have pronounced signs of volume contraction. Sodium chloride tablets and fludrocortisone may be helpful in less severe cases with adequate volume repletion [51]. Hypotonic fluids, free water, diuretics, and antidiuretic hormone therapy (vasopressin and desmopressin) should be avoided. Early use of fludrocortisone has been shown to reduce natriuresis [64] and the risk of vasospasm in hyponatremic SAH patients [46, 87].
On the other hand, free water restriction is required and often sufficient to treat SIADH and many patients have self-limiting disease . Identification and treatment of the underlying cause is an essential step. The volume of fluid restriction can be determined using the ratio of combined urinary sodium and potassium divided by serum sodium but patients are typically restricted to 1–1.5 L/day in clinical practice.
Some medications are effective as adjunct therapy to fluid restriction. A first choice is urea, an osmotic diuretic that enhances water flow from tissues into the interstitial fluid and plasma, thereby increasing serum osmolality. Demeclocycline, a tetracycline antibiotic, is also effective but can induce insipidus diabetes. More recently, vasopressin receptor antagonists (VRA) have emerged as a new class of drugs for the treatment of euvolemic and hypervolemic hyponatremia [88]. These molecules antagonize V2 receptors in the distal nephron, inducing then an aquaresis. Their optimal place in the treatment algorithm remains to be determined [51].
The treatment of hyponatremia-induced seizures and SE beyond correction of serum sodium levels has not been formally studied. Isolated seizures probably do not warrant any specific intervention but anti-seizure medications may be administered in case of SE. It is also probably reasonable to avoid medications that can aggravate hyponatremia, such as oxcarbazepine, carbamazepine, and valproic acid .
Hypernatremia
Hypernatremia is defined by a serum sodium concentration >145 mM/L. It is less frequent than hyponatremia with an incidence of 10% [89] of hospitalized patients.
Between 3.6 and 17% of critically ill patients experience hypernatremia with a sodium concentration >150 mM/L [39, 40, 43, 48, 90], most often during their first week in the ICU [40, 91].
It is often perceived as an indicator of the severity of the underlying disease and of the quality of care [92] and is associated with an increased risk of mortality [93].
Hypernatremia is invariably associated with hyperosmolality [94] but can result from a net loss of free water or an excess of sodium intake or retention, and thus can be either hypovolemic or hypervolemic, respectively (Table 18.4).
Table 18.4
Cause of hypernatremia
Hypovolemia | • Altered thirst mechanism • No access to water • Intra-renal water loss: central or nephrogenic diabetes insipidus, osmotic diuresis, diuretics • Extra-renal water loss: respiratory or GI losses, fever |
Hypervolemia | • Iatrogenic: administration of large amounts of fluids to avoid hypovolemia • Mineralocorticoid excess: primary hyperaldosteronism, Cushing syndrome |
Hypovolemic hypernatremia is most often due to water deficiency or inadequate water supplementation. It is most common in young children, the elderly, and critically ill individuals who rely on others for their water intake. Fever, GI losses, and the use of diuretics are common precipitants.
Diabetes insipidus (DI) is defined by an inappropriate free water outflow with polyuria. It is the consequence of the failure of release of ADH (central DI) or of the resistance of the kidney to ADH (nephrogenic DI). The impermeability of the collecting ducts to water due to the lack of ADH activity results in urine dilution (<100 mOsm/kg), while plasma osmolality rises as a consequence of the progressive dehydration. Causes of DI are listed in Table 18.5. The severity of DI depends of the rate of residual ADH secretion. It is particularly dangerous if the thirst mechanism is impaired too, which can occur with central DI due to a cerebral mass in the hypothalamus-pituitary region. Central diabetes insipidus occurred in 3.7% patients in a neurosurgical intensive care unit [42].
Table 18.5
Causes of diabetes insipidus
Primary polydipsia (psychogenic polydipsia) Central (neurogenic) diabetes insipidus • Idiopathic, possibly autoimmune • Pituitary surgery • Traumatic brain injury • Anoxic encephalopathy • Death by neurological criteria (brain death) • Hereditary Nephrogenic diabetes insipidus • Hereditary (ADH receptor V2 mutation) • Lithium • Hypercalcemia |
Critically ill patients receiving mechanical ventilation are inclined to develop hypernatremia. Iatrogenic sodium gain occurs quickly with hypertonic perfusions but isotonic solutions can also lead to hypernatremia in the presence of diluted urines [95]. Potassium-rich solutions can also trigger sodium accumulation [91]. Diuretics, and in particular loop diuretics, hyperglycemia, osmotic diuresis, acute and chronic renal dysfunction, nasogastric drainage, diarrhea, and fever are all common in critical care patients. They induce the loss of free water and can further aggravate the hypernatremia.
Symptoms and signs of hypernatremia include nausea, headache, lethargy, and ultimately coma. Seizures are infrequent but can occasionally be seen in the setting of extra-pontine demyelination, which occurs with acute severe hypernatremia [96, 97].
Given the rarity of seizures attributable to hypernatremia, alternate causes should be actively sought, such as cerebral sinus or cortical vein thrombosis [98, 99]. In fact, seizures are much more likely to occur as the consequence of the aggressive treatment of chronic hypernatremia than as the consequence of hypernatremia itself [100, 101]. It is important to remember that it is the rate of decrease in serum sodium concentration itself rather than the absolute value that determines the risk of seizure.
Another factor confounding the relationship between hypernatremia and seizures is that generalized tonic-clonic seizures are accompanied by water shift into the intracellular space and can cause transient hypernatremia [102].
Management of Hypernatremia
The etiology of hypernatremia can usually be determined by concomitant measurement of serum and urine osmolality. The diagnosis of central DI is suggested by the presence of hypotonic urine (<300 mM/kg) in the setting of an elevated serum osmolality (>295 mM/kg). Central DI can be distinguished from nephrogenic DI by fluid restriction and desmopressin response tests. A magnetic resonance imaging is indicated to identify the underlying etiology of central DI.
Urine osmolality >600 mM/kg indicates intact ADH secretion and action and suggests either dehydration from renal, GI or insensible loss, or sodium overload. These causes can be further discriminated by measuring the urine sodium concentration, which is typically <25 meq/L in case of volume depletion and >100 meq/L in case of sodium overload.
Symptomatic management of hypernatremia begins by assessing the degree of water deficit and determining the appropriate rate of correction. Water deficit can be estimated using the following formula:
![$$ \begin{array}{c} Water\ deficit= current\ total\ bodyweight\times \\ {}\kern1.5em ( serum\left[ Na\right]/140-1).\end{array} $$](https://i0.wp.com/neupsykey.com/wp-content/uploads/2017/08/A145499_3_En_18_Chapter_Equb.gif?w=960)
![$$ \begin{array}{c} Water\ deficit= current\ total\ bodyweight\times \\ {}\kern1.5em ( serum\left[ Na\right]/140-1).\end{array} $$](https://i0.wp.com/neupsykey.com/wp-content/uploads/2017/08/A145499_3_En_18_Chapter_Equb.gif?w=960)
In patients with chronic hypernatremia (present for longer than 48 h), the goal is to lower the serum sodium by10 meq/L in 24 h, since lowering the plasma sodium concentration too rapidly can lead to cerebral edema and seizures. There is also evidence that excessively slow correction (lower than 6 meq/L in 24 h) is detrimental as well, since untreated severe hypernatremia can cause permanent brain injury.
The amount of water to be replaced in the first 24 h is limited and can thus be estimated as:
![$$ \begin{array}{c} Desired\ water\ replacement= Water\ deficit\times \\ {}\kern1em (10)/( Serum[ Na]-140).\end{array} $$](https://i0.wp.com/neupsykey.com/wp-content/uploads/2017/08/A145499_3_En_18_Chapter_Equc.gif?w=960)
![$$ \begin{array}{c} Desired\ water\ replacement= Water\ deficit\times \\ {}\kern1em (10)/( Serum[ Na]-140).\end{array} $$](https://i0.wp.com/neupsykey.com/wp-content/uploads/2017/08/A145499_3_En_18_Chapter_Equc.gif?w=960)
In patients with known acute hypernatremia (present for 48 h or less), correction can occur faster and the entire water deficit can be corrected in 24 h.
These methods only measure the current deficit. The fluid repletion regimen should also take into account any ongoing losses; otherwise the correction rate will be substantially lower than expected.
Serum sodium has to be measured 2–4 h after initiation of the repletion regimen and the correction rate adjusted accordingly. Once a serum sodium concentration of 145 meq/L is reached, the infusion rate can be decreased to 1 mL/kg/h until normonatremia is reached.
Free water is usually provided as an intravenous solution of 5% dextrose in water. In patients who can drink or have nasogastric tubes, free water can be administered enterally every 4–6 h. Many hypernatremic patients have concurrent volume depletion and will require fluid resuscitation with 0.45% or 0.225% saline solution, which provide only one half or three-quarters, respectively, of free water, compared to dextrose solution. This needs to be taken into account when estimating the required volume of fluid needed to correct serum sodium levels.
Similarly, many patients also have hypokalemia. Potassium can be added to the administered fluids but thus will also decrease the amount of free water being given.
The rapid administration of 5% dextrose solution can lead to hyperglycemia, especially in patients with diabetes mellitus or a physiological stress. In order to avoid increased water losses from glycosuria, a 2.5% dextrose solution may be preferred in patients who develop hyperglycemia.
In addition to symptomatic management, patients with DI require desmopressin therapy.
Calcium and Seizures
Calcium Homeostasis
Serum calcium plays an essential role in skeletal mineralization, neuromuscular excitability, and coagulation. Intracellular calcium is involved in muscular contraction, hormone secretion, nerve conduction, and cell proliferation .
Approximately 90% of total body calcium is stored in bones. Calcium in the blood is bound to proteins, mainly albumin, and small anions such as phosphate and citrate. The active fraction is extracellular ionized calcium that represents only 1 mM. Intracellular calcium is mainly bound to proteins or sequestered in organelles.
Calcium balance is regulated mainly by the interplay between parathyroid hormone (PTH) and calcitonin, secreted by parafollicular cells in the thyroid. Ionized serum calcium exerts a feedback control on the parathyroid gland and parafollicular cells through calcium-sensing receptors so that PTH is released and calcitonin secretion is inhibited when serum calcium level decreases and PTH release is inhibited and calcitonin is released when calcium level increases.
PTH increases calcium concentration by reducing urinary calcium and phosphate excretion, increasing bone resorption and stimulating the synthesis of calcitriol (1,25 dihydroxyvitamin D3), the activated form of vitamin D3, in the kidney. Calcitriol stimulates calcium resorption by the gut. Calcitonin decreases calcium levels by stimulating bone mineralization.
Hypocalcemia
The most common cause of hypocalcemia is renal failure. (Table 18.6). The gastrointestinal absorption is reduced because of decreased renal production of calcitriol and because of increased serum phosphorus.
Table 18.6
Causes of hypocalcemia
Etiologies |
---|
Renal failure |
Hypoparathyroidism or pseudohypoparathyroidism (PTH resistance) |
Magnesium deficiency |
Pancreatitis |
Osteoblastic metastasis |
Tumor lysis syndrome |
Sepsis |
Vitamin D deficiency or receptor defects (nutritional deficiency, malabsorption) |
Calcium-sensing receptor mutations |
Drugs: bisphosphonates, anticonvulsants, calcitonin, phosphate, tyrosine kinase inhibitors (Imatinib) |
Hypoalbuminemia (pseudohypocalcemia) |
Transfusion of citrated blood products |
Hyperphosphatemia |
Hypoparathyroidism is the second most important cause of hypocalcemia and can be the consequence of surgery, an autoimmune disease (autoimmune polyglandular syndromes), hypomagnesemia, radiation, infiltrative diseases, or a genetic disorder. Up to 54% patients with hypoparathyroidism may present with seizures [103, 104].
Hypocalcemia is common in critically ill patients [105]. Sepsis and severe illness can lead to hypocalcemia by several mechanisms: hypomagnesemia, serial blood transfusions, acute renal failure from inflammation, and kidney injury [106]. The prevalence is variable but can reach 55–85% [107–109]. Hypocalcemia correlates with disease severity and is associated with an increased mortality in many studies [106–111]. Iatrogenic hypocalcemia can be accompanied by seizures. Drugs that have been implicated include proton-pump inhibitors [112, 113], bisphosphonates [114, 115], calcitonin, and tyrosine kinase inhibitors (imatinib). Proton-pump inhibitors reduce magnesium absorption by the gut and may lead to hypomagnesemia and secondary hypocalcemia . Accidental parathyroidectomy during thyroidectomy can result in unexpected and untreated hypocalcemia and seizures, sometimes years after the surgery [116–118].
Hypocalcemia-related seizures are well documented in children with congenital or idiopathic hypoparathyroidism [119–122], celiac disease [123], and rickets [124].
Neonates are at particular risk of hypocalcemia, either during the first 3 days after birth, when it is associated with premature birth, hypoxic-ischemic encephalopathy and maternal diabetes, or after the first week, when it is caused by vitamin D deficiency, dietary phosphate overload, and endocrine disorders [125]. Neonates born from mothers with asymptomatic hyperparathyroidism may develop impaired parathyroid responsiveness to hypocalcemia and seizures [126]. Hypocalcemia-related neonatal seizures used to be frequent [127] but their incidence has steadily decreased, owing to better screening and prevention of hypocalcemia in high-risk neonates.
Finally, the anticonvulsant phenytoin may increase the metabolism of vitamin D, leading to hypocalcemia and occasionally to hypocalcemia-related seizures [128]. Therefore, clinicians are advised to check calcium in cases of anti-epileptic drug refractory seizures.
Normal total serum calcium ranges between 8.5 and 10.2 mg/dL and ionized calcium between 4.4 and 5.4 mg/dL (1.1 and 1.35 mM/l).
In case of protein depletion, total calcium decreases but the ionized calcium, which is metabolically active, is normal because it is still hormonally regulated. This situation is called pseudohypocalcemia . Corrected serum calcium levels can be estimated using the following formula:
![$$ Corrected\left[ Ca\right]= Measured\left[ Ca\right]+0.8\times \left(4-\left[ albumin\right]\right). $$](https://i0.wp.com/neupsykey.com/wp-content/uploads/2017/08/A145499_3_En_18_Chapter_Equd.gif?w=960)
![$$ Corrected\left[ Ca\right]= Measured\left[ Ca\right]+0.8\times \left(4-\left[ albumin\right]\right). $$](https://i0.wp.com/neupsykey.com/wp-content/uploads/2017/08/A145499_3_En_18_Chapter_Equd.gif?w=960)
Similarly, ionized calcium levels can be estimated from total calcium levels:
![$$ \left[ C{a}^{2+}\right]= Total\left[ C a\right]-0.8\times \left[ protein\right]. $$](https://i0.wp.com/neupsykey.com/wp-content/uploads/2017/08/A145499_3_En_18_Chapter_Eque.gif?w=960)
![$$ \left[ C{a}^{2+}\right]= Total\left[ C a\right]-0.8\times \left[ protein\right]. $$](https://i0.wp.com/neupsykey.com/wp-content/uploads/2017/08/A145499_3_En_18_Chapter_Eque.gif?w=960)
However, the binding of the albumin-calcium complex is influenced by pH, which renders the estimate inaccurate in most critically ill patients where direct measure of ionized calcium should be preferred. For instance, a study in trauma patients compared 22 methods to estimate corrected total serum calcium and serum ionized calcium and found that they had an average sensitivity of 25% for the detection of hypocalcemia [129]. However, a marked serum hypocalcemia with less than 7 mg/dL total calcium levels is generally a good marker of ionized hypocalcemia in critically ill patients [130].
Manifestations of hypocalcemia are explained by the hyperexcitability of the peripheral and central nervous system. Tetany is the hallmark of acute hypocalcemia, mostly occurring with ionized calcium below 4.3 mg/dL and total serum calcium below 7.5 mg/dL [131]. Patients first complain of paresthesias around the mouth and in the extremities and of muscle spasms. The Chvostek and Trousseau signs can be observed. Gastrointestinal symptoms can occur because of the stimulation of the vagal nerve system, with dysphagia, nausea, biliary colic, abdominal pain, or cramping [19, 132]. Lethargy, stupor, and finally coma occur. Laryngospasm and bronchospasm are potential and severe complications.
Chronic hypocalcemia, mostly seen in patients with hypoparathyroidism, leads to skin and dental changes, basal ganglia calcifications, extrapyramidal signs (parkinsonism or choreo-athetosis), and cataract.
Seizures are most commonly of the generalized tonic-clonic type but nonconvulsive status epilepticus can occur [133]. Patients often exhibit symptoms and signs of neuromuscular hyperexcitability but isolated seizures have been reported [134].
Management of Hypocalcemia
Symptomatic patients with tetany or seizures and patients with prolonged QT interval or with calcium below 7.5 mg/dL should receive intravenous calcium. Otherwise, oral supplementation can be used.
Calcium gluconate is generally given diluted at a dose of 1 or 2 g over 10–20 min and not faster because of the risk of cardiac dysfunction. The supplementation is continued with an intravenous drip of 10% calcium gluconate or calcium chloride. Calcium gluconate is usually preferred, as it carries a lower risk of tissue necrosis in case of accidental extravasation. The infusion rate is guided by improvement of symptoms and ionized serum calcium monitoring every hour.
The underlying cause should be identified, by measuring PTH, magnesium, creatinine, phosphate, and vitamin D levels, and treated. Concurrent hypomagnesemia should be corrected.
Hypercalcemia
The most frequent causes of hypercalcemia are primary hyperparathyroidism, due to parathyroid adenoma, and malignancy. Rare causes include granulomatous diseases, such as sarcoidosis or tuberculosis, drugs, and genetic disorders. A mild chronic hypercalcemia is typical of primary hyperparathyroidism and is often asymptomatic while the hypercalcemia associated with malignancy is usually more severe (>13 mg/dL), develops more rapidly and is thus more often symptomatic.
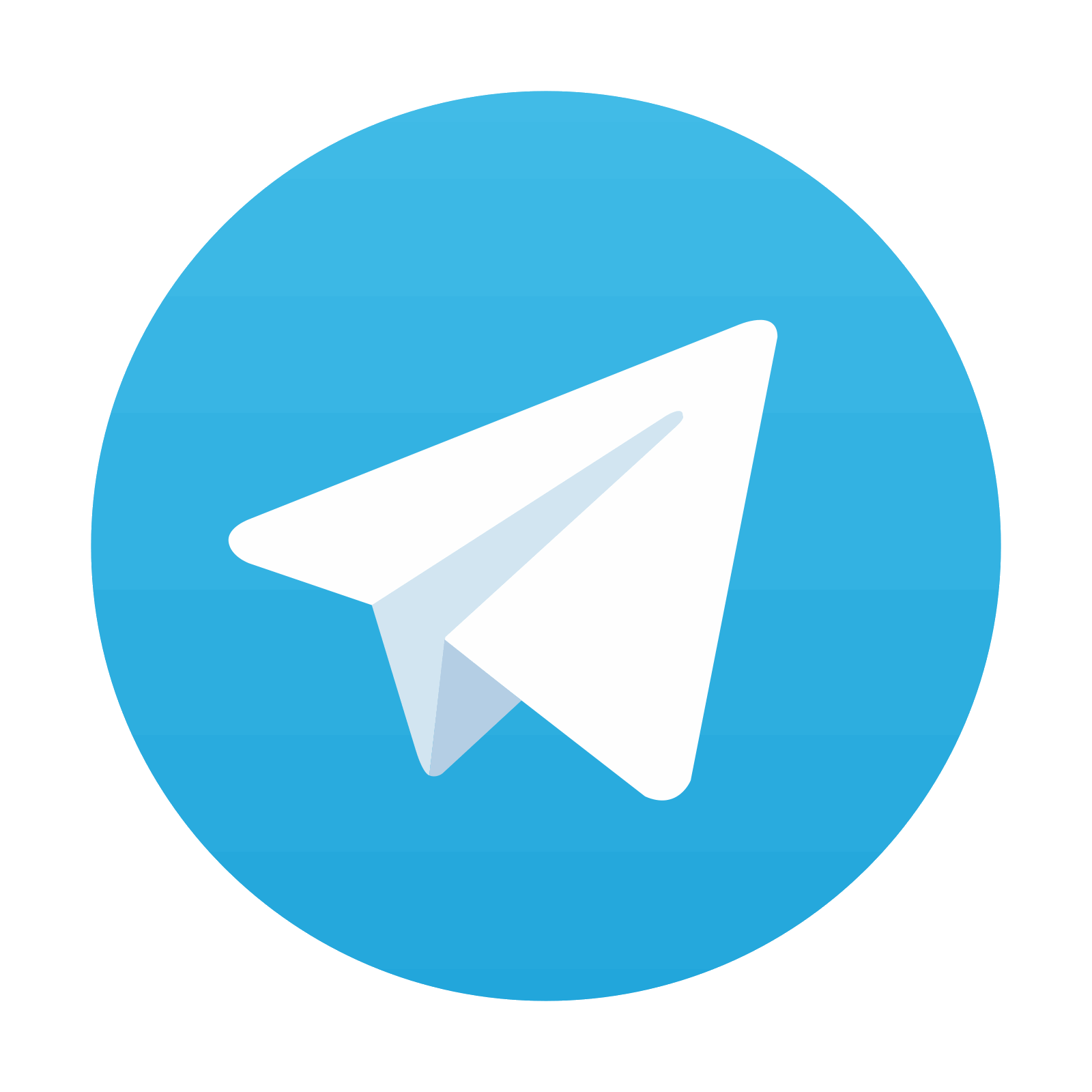
Stay updated, free articles. Join our Telegram channel
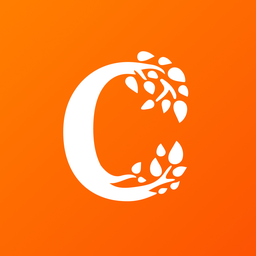
Full access? Get Clinical Tree
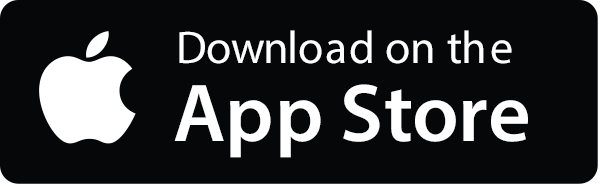
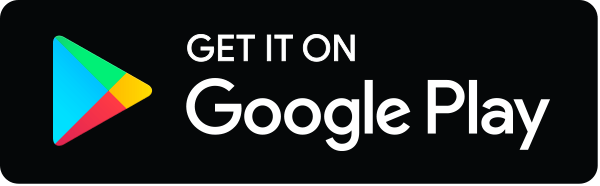