INTRODUCTION
Startling advances in medicine have extended our lives and given us healthier bodies, but not healthier minds. The mere fact that we are living longer consigns more of us to enduring our final years ravaged by diseases like Alzheimer’s or stroke, and suffering all the psychiatric manifestations that are part and parcel of these diseases. Currently available treatments do little. Therapies that replace the damaged brain tissue, either by regeneration or by transplantation, are needed. Unfortunately, both a poor theoretical understanding of neuronal generation/regeneration, and a lack of practical tools to effectively deliver these genes to the right cells, in the right amounts, continue to hamper the field of regenerative medicine. Similarly, harnessing the therapeutic potential of stem cell transplants remains a promise unfulfilled because neural stem cells (NSCs) simply do not function properly when transplanted into regions other than those that normally support adult neurogenesis. Worse yet, even those few privileged environments that normally support neurogenesis are rendered inhospitable to newly implanted NSCs by many of the same conditions that make someone a candidate for neural transplantation in the first place.
The idea of transplanting neurons to repair damaged brain is not new; the first trials, using foetal tissue, in Parkinson’s patients were performed more than two decades ago. Initial optimism gave way to the harsh reality that this was not the cure many had hoped for. Many hypothesized that more mature cells simply had lost the ability to learn how to adapt to their new host environment. Naturally, waves of enthusiasm have heralded discoveries from foetal NSCs to human embryonic stem cells (hESCs), with their uncanny pluripotency. Still, somatic cell therapy remains an elusive prospect because transplanted cells do not properly engraft, either failing to properly differentiate, dying or not integrating into the local circuitry. Developing therapies that provide real clinical improvement requires leveraging our newfound appreciation of the microenvironment within which neurons normally develop.
Since the field of neural repair remains in its infancy, any review of this field (including this one) must contain a fair degree of educated guessing about the future, some of which will turn out to be wrong. To minimize the impact this might have, we will focus on communicating the essentials of current thinking in the field, rather than trying to divine what approach(es) will ultimately be successful. To this end we have broken this chapter down into three sections. The first section aims to supply the reader with a historical understanding of early clinical repair trials, highlighting some of their limitations and questions raised. The second section provides a primer on the regenerative capacity of the adult brain. In this section we introduce the concept of an adult stem cell niche. To the clinician, some of these sections may seem technically dense; we have made every attempt to minimize the use of unnecessary jargon. However, the reader should recognize that an under-appreciation of the importance of the niche goes a long way towards explaining the shortcomings of previous trials. Conversely, understanding how disease or ageing alters the functioning of these microenvironments will be absolutely critical for understanding the rational of any future neuroregenera- tive therapy trials. The third section introduces the clinical reader to some of the current and emerging tools at the gene therapist’s disposal. Finally, we will provide some plausible scenarios where the discussed concepts and tools can be integrated to produce workable future clinical trials. In sum, this chapter should empower the reader with the necessary framework for understanding ongoing developments, rather than trying to divine which approaches will ultimately be successful.
BIRTH OF REGENERATIVE MEDICINE
Molecular strategies for functionally repairing the damaged brain fall into one of three categories: (i) replace/repair circuitry; (ii) potentiate function of remaining cells; and (iii) halt disease progression1. The cell replacement strategy has the longest track record with the first foetal neuron transplants being reported in 19892. The lion’s share of transplantation trials was performed on patients suffering from either Huntington’s or Parkinson’s disease. However, the technique has also been applied to patients with macular degeneration, stroke and, most recently approved, children with Batten’s disease. More recently, there has been some forays into gene-therapy-based approaches. Below we will review the finding from these previous transplantation trials, with an emphasis on calling out those features that are amenable to improvement in future studies.
Parkinson’s Disease
Parkinson’s disease (PD) is characterized by relatively selective loss of the dopaminergic neurons in the nigrostriatal tract, though all brain regions are ultimately affected in later stages. Nigrostriatal degeneration results in a well-characterized syndrome of progressive rigidity, bradykinesia, tremors, poor gait/balance, and later dementia in many patients. The first human neural transplants involved unilateral stereo- tactic injection of mesencephalic dopamine neurons, obtained from 7 to 9 weeks gestational age human foetuses, into the putamen of long-term Parkinson’s patients2-11. These trials delivered initially encouraging results. Imaging studies (PET or MRI) revealed graft viability and recipients exhibited motor improvement, with reduced medication doses.
Because initial trials were all unblended, concerns over the placebo effect prompted more sophisticated trials. These included sham surgery and blinded rating of imaging results. In a larger trial reported by Freed and colleagues12 40 PD patients, ranging in age from 34 to 75 years old, received either embryonic mesencephalic neuroblasts/neurons or sham surgeries and were followed, double-blind, for the succeeding 12 months. They found that although the transplanted cells survived transplantations, the procedure only seemed to clinically benefit younger (younger than 60 years of age) PD patients. Unfortunately, the more carefully this promising therapy was evaluated, the less ‘promising’ it appeared13. Some patients developed uncontrollable dyskinesia, presumably due to aberrant axon regrowth; many showed limited improvement. However, this approach has fallen out of favour because it neither slows disease progression, not is it more effective than aggressive medication, and probably less effective than deep brain stimulation.
With a guide to designing future therapies, it is interesting to note that on analysis some transplanted neurons developed Lewy bodies14‘15. This finding suggests that merely placing new tissue into an inhospitable (deteriorating) brain is not a viable solution. The most recent regenerative trials for PD have focused on changing this environment by providing additional trophic support. Specifically, there have been attempts to augment glial-cell-derived growth factor (GDNF) levels in the striatum, as this protein has shown a significant ability to protect dopaminergic neurons from a variety of experimental insults. The farthest along trial involved direct infusion of GDNF into the putamen16. The preliminary results of these studies are again promising, but only time will tell16-18. The current trend in PD trials is towards use of adeno-associated virus (AAV) to deliver and induce production of the therapeutic factor (neurturin, a GDNF-like ligand19) or enzyme (glutamic acid decarboxylase20; aromatic l-amino acid decarboxylase21).
Alzheimer’s Disease
In constrast to PD, only one group has made any progress towards applying regenerative medicine to Alzheimer’s patients. The two trials differed in their technical approach, but shared the goal of delivering nerve growth factor (NGF), a survival factor for cholin- ergic neurons, to the brain regions suffering cholinergic neuron loss. Using an ex vivo gene therapy approach, they implanted fibroblasts that were engineered to secrete NGF22. The other study used in vivo gene therapy, utilizing AAV to induce local NGF production23. The initial results seem promising, but the long-term benefit and safety is an open question.
Huntington’s Disease
Huntington’s disease (HD) serves as a model neuropsychiatric disorder, despite being a relatively rare disease (7 per 100 000 persons). A toxic gain-of-function mutation (polyglutamine trinucleotide repeat expansion) in the huntingtin protein causes this dominantly inherited movement disorder. Huntingtin is widely expressed throughout the brain, resulting in personality changes or other psychiatric manifestations that may precede overt motor symptoms by almost a decade. However, it is the striking degeneration of the striatum that burdens patients with its characteristic choreoathetoid movements. Eventually, all patients will suffer major psychiatric symptoms, and if they live long enough, dementia will ensue (DSM-IV; 294.1 Dementia Due to Huntington’s Disease).
Transplantation trials in HD were contemplated soon after the purported ‘success’ of foetal neural transplant in PD patients. These open label trials (i.e. no sham surgery) entailed transplantation of committed neuroblasts from foetal human striatum into the brains of moderately affected HD patients24,25 (and later26,27). The results of these trials appeared mixed. Bachoud-Levi and colleagues reported that on two-year follow-up, three out of five transplant recipients exhibited improvement of both motor and cognitive symptoms28,29. These improvements correlated recovery of metabolic activity in the specific striatal location of the transplants, as well as connected regions of the cortex. In contrast, another group following seven patients used PET to examine graft survival. They found evidence of poor graft survival/function. Moreover, they could not demonstrate a significant relationship between changes in PET and clinical function, forcing them to conclude that there was no benefit from transplantation30.
On longer-term follow-up, Bachoud-Levi and colleagues found mixed results with regard to improvements in motor symptoms. Among those patients who displayed an improvement at two years, chorea remained stable at an improved level for the following four years31. While chorea was improved, dyssomnia became steadily more prominent, paralleling the results in PD transplant trials. More encouraging were their observations of limited cognitive decline among initial responders. They showed no worsening on the Mattis dementia rating scale and only slight worsening on the Trails A. Although the relatively small numbers of these follow-up studies makes it difficult to draw any major conclusions, they suggest that transplants in HD may be more beneficial than in PD. Unlike PD, there are very few pharmacological interventions for HD, making an invasive procedure like cell transplant more appealing. Still, it seems apparent that transplantation is not going to become more pervasive until concomitant neuroprotective treatment becomes available. On a more general cautionary note, there are at least two documented cases of tumour formation among patients who have received cell transplants32,33, raising the spectre of additional safety concerns.
REGENERATIVE CAPACITY OF ADULT BRAIN A Brief History of Adult Neurogenesis
For nearly the entire twentieth century the regenerative capacity of the adult brain was considered to be almost nil. It was dogma that the number of neurons in the adult mammalian brain was fixed near birth. This sentiment was reflected by the great neuroanatomist Santiago Ramon y Cajal, who wrote, ‘Once development was ended, the fonts of growth and regeneration of the axons and dendrites dried up irrevocably. In adult centers, the nerve paths are something fixed, and immutable: everything may die, nothing may be regenerated’34. As early as 1897 there was evidence for undifferentiated cells in the adult brain that had the potential to become either neurons or glia; however, this view was not accepted for almost another century. (Charles Gross provides a fascinating historical accounting of this cautionary tale of scientific sophistry and denial35.)
In the early 1990s, Reynolds and Weiss demonstrated that one could easily isolate multipotent progenitors from the adult mouse striatum. These precursors expanded in culture by application of epidermal growth factor (EGF) and then directed towards differentiating into neurons or glia36‘37. More than anything, the ease with which other laboratories could replicate their findings ushered in the acceptance of adult neurogenesis. Subsequently, Eriksson and colleagues examined the postmortem brains of cancer patients who received BrdU as part of their chemotherapy regimen. They found that this thymidine analogue, which is incorporated into replicating DNA, was present in adult borne hippocampal neurons38. This elegant study was later extended and replicated, demonstrating a similar neurogenic zone in the subventricular zone (SVZ), neatly paralleling previous animal studies39-41.
The lingering question of why all mammals retain this form of plasticity has yet to be answered satisfactorily. That the hippocampus plays a critical role in short-term memory formation and is implicated in emotional regulation suggests that adult neurogenesis plays an evolutionarily conserved role in these processes. The most straightforward evidence suggests that adult neurogenesis plays a role in memory formation. For example, the two most potent stimulators of adult neurogenesis, exercise and environmental enrichment, increase hippocampal neurogenesis and improve performance on cognitive tasks like the Morris water maze, even among aged animals. These improvements are only seen with tasks that are hippocampus-dependent (e.g. the water maze), but not with hippocampus-independent learning (e.g. active shock avoidance or delayed eye blink) (reviewed by 42-45).
Neural Stem Cells and Their Environment What is a neural stem cell?
Throughout our lifetime new neurons are generated from nascent precursors termed neural stem cells. ‘Stem cells’ have garnered tremendous popular and scientific attention over the past several years, but this raises the question of what is a neural stem cell? Since the discovery and acceptance of adult neurogenesis over 10 years ago, the field has been mired by confusing language and inconsistent definitions of what it means to be a neural stem cell46. In order to avoid such confusion, the following definitions will apply throughout this chapter: a neural stem cell is one that self-renews and has the potential to give rise to multiple cell lineages. Precursors (e.g. adult hippocampal precursor; AHP) are proliferating multipotent cells that do not self- renew. It is often difficult to experimentally distinguish NSCs from multipotential precursors. Therefore, in the absence of data showing that a cell truly self-renews, it is more conservative to refer to multipotent cells as precursors, and not stem cells. In contrast, lineage committed progenitors (e.g. neural progenitors) are rapidly proliferating cells whose differentiation potential is limited to one cell type.
Emergence of adult NSCs
The earliest NSCs begin their life as a thin layer of symmetrically dividing neuroepithelium lining the neural tube. In later embryonic development, these neuroepithelia become radial glia, which support the migration of migrating neuroblasts. These radial glia persist in the neonatal brain and ultimately transform into an astrocytic subtype with the properties of an NSC47. These NSC appear to constitute two preserved germinal centres in the adult mammalian brain, one in the SVZ and the other in the subgranular zone (SGZ) of the hippocampal dentate gyrus. The former migrate through the rostral migratory stream (RMS) to take up residence in the olfactory bulb, while the latter migrate a short distance to form the dentate granule cells47.
Neurogenic stem cell niche
The anatomy of these two neurogenic region shares many similarities. Both constitute thin strips containing small clusters of proliferating cells (types A/G, B, C/D) in close opposition to vascular endothelium48,49. The type B cells are slowly proliferating, self- renewing astrocytes that are the real NSCs in these regions. The C (SVZ) and D (SGZ) cells are more rapidly dividing neural progenitors (transit amplifying), while the A (SVZ) and G (SGZ) cells are the immature neurons. The intricacies of the anatomy and the developmental process are far too complex for this discussion and are reviewed extensively elsewhere47,49. What is important is to recognize that the NSCs, their progeny and their blood supply reside in close opposition, creating a somewhat self-contained microenvironment. This specialized milieu is termed the stem cell niche50.
Three compartments comprise this niche: ultrastructure, cells and milieu. (i) Ultrastructure: extracellular matrix and other cellular scaffolding maintain the gross cytoarchitecture of specific brain regions. Since guidance molecules are immobilized on these scaffolds, their loss can cause aberrant dendritic arborization and impaired axonal pathfinding of transplanted/regenerating neurons. (ii) Cellular components include NSCs, astrocytes (supportive and immunological), neurons, oligodendrocytes, microglia, and vascular endothelium and smooth muscle. (iii) The milieu is a metastructure composed of the many dynamically regulated, diffusible factors released by the constituent cells. Gradients of these factors are responsible for coordinating: (i) homing of immune cells; (ii) migration of neuroblast during foetal development (or transplanted neural stem cells); and (iii) neuritogenesis and synaptogenesis.
Niche Function
The niche, like a womb, creates a uniquely nurturing environment to support the birth and development of NSCs into full-fledged neurons. In addition to this supportive function, it serves to insulate the NSCs from the greater milieu. The constituent cells create this environment and generate the milieu, and in turn, each is impacted by it. Therefore, no cell is an island (with apologies to John Donne), the fate of each intimately affected by its neighbours. In toto, the niche preserves the delicate balance between NSC maintenance and differentiation and directs lineage decisions.
How is the niche regulated?
The SVZ/SGZ niche contains cells in various stages of development, from stem cells to committed progenitors to maturing neurons, as well as vasculature and ependyma (SVZ). As such, the developing NSCs are subjects to a host of intrinsic (e.g. neighbouring cells) and extrinsic (e.g. from blood or CSF) cues. These ‘niche players’ represent the prime targets of proposed gene therapy protocols. Formally, there are three forms of signalling at play: (i) externally derived signals conveyed via the blood (endocrine) or CSF (exocrine); these include diffusible factors like muscle-derived IGF- 1 or VEGF, or inflammatory cytokines (e.g. interleukin-6, IL-1β or tumour necrosis factor alpha; TNFa); (ii) paracrine factors produced by local astrocytes or neurons (e.g. BDNF, FGF-2, Wnt, neuro- transmitters); and (C) cell-autonomous signalling, which includes autocrine factors like Wnt or PDGF as well as genetically preprogrammed differentiation51-53.
Table 55.1 Niche targets for future gene therapy trials
NSC, neural stem cell; NPC, neural progenitor; OPC, oligodendrocyte progenitor.
a Used in clinical trials.
bFDA approved (alternate indication).
Secreted proteins | Niche effects |
Bone morphogenic protein4 [BMP4] | Astroglial differentiation |
Brain derived neurotrophic factor [BDNF]a | NPC/neuron survival |
Ciliary neurotrophic factor [CNTF]a | Astroglial differentiation |
CCL5 (Rantes) | NSC quiescence |
CXCL12 (SDF1α) | NPC chemoattractant, NSC |
CX3CL1 (Fractaline) | NPC differentiation |
Epidermal growth factor [EGF] | NSC/NPC survival and proliferation |
Erythropoietinb | NPC differentiation |
Fibroblast growth factors [FGF-2/FGF-20]a | NPC proliferation |
Glial-derived neurotrophic factor [GDNF]a | Neuron survival |
Granulocyte macrophage colony stimulating factor [GM-CSF]b | Neuronal differentiation |
Hepatocyte growth factor (HGF)a | NPC/neuron survival |
Insulin-like growth factor 1 [IGF-1]b | NPC proliferation, neuron survival |
Leukaemia inhibitory factor [LIF] | NSC proliferation and survival |
Neurotrophin 3,4/5 [NT3, NT4/5] | Neuron survival |
Nerve growth factor [NGFa]a | Neuron survival |
Platelet-derived growth factor [PDGF]b | OPC proliferation and survival |
Progranulin [PGRN] | Neuronal survival (?), anti-inflammatory(?) |
R-spondin [RSPOa] | NPC proliferation (?) |
Transforming growth factor beta [TGFβ] | Neuronal differentiation, anti-inflammatory |
Vascular endothelial growth factor [VEGF]a | Angiogenesis, NPC/neuron survival |
Wnt3,Wnt7a, Wnt8b | NSC/NPC proliferation and survival |
Wnt5a, Wnt7b | Neuronal differentiation |
Table 55.1 summarizes some of the most prominent modulators of the adult NSC niche; however, the full panoply of secreted factors influencing the niche is far too extensive for the confines of this chapter (for review see54-62).
‘Normally’ aging niche
Neurogenesis reduces by more than 50% with advancing age. Some of the non-mutually exclusive hypotheses put forth to explain this observation include (i) functional, active downregulation63; (ii) inflammation64; (iii) burnout/depletion of stem cells (e.g. secondary to reduced Wnt61); (iv) niche changes that directly suppress trophic support65; (v) reduced circuit activity, which in turn could decrease BDNF/trophic support66; (vi) elevated cortisol, which accompanies normal ageing63; (vii) reduced physical activity, which reduces IGF-1, again, limiting trophic support67-71; and (viii) less intellectual stimulation might functionally reduce need for neurogenesis or might limit circuit activity indirectly (intellectual reserve hypothesis72
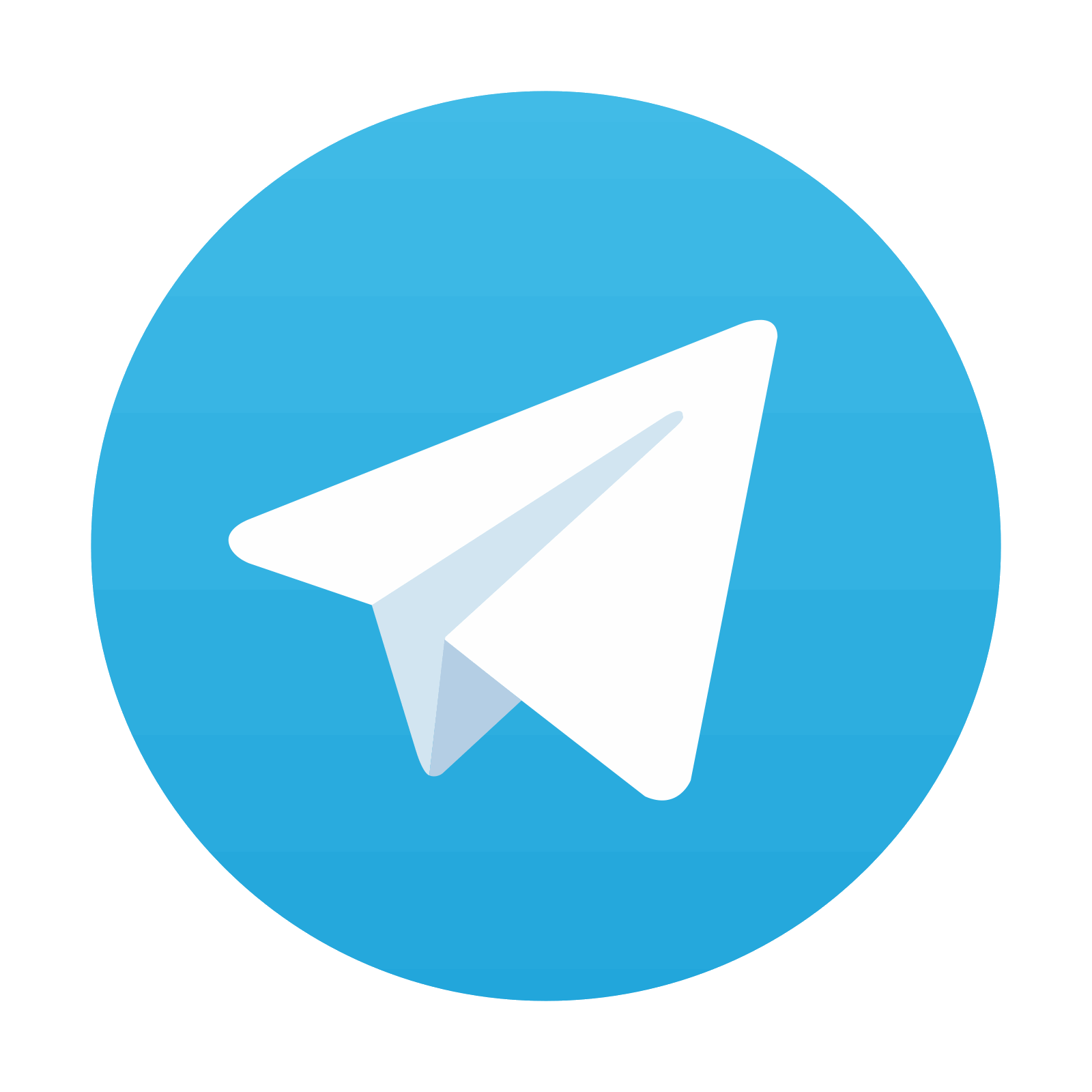
Stay updated, free articles. Join our Telegram channel
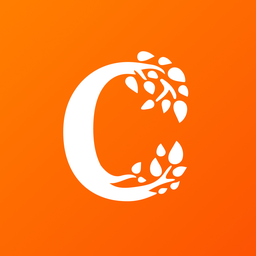
Full access? Get Clinical Tree
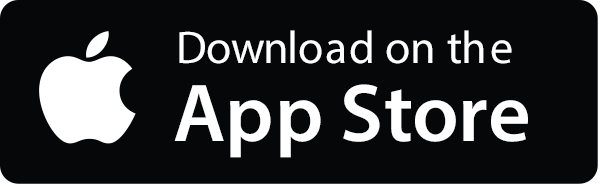
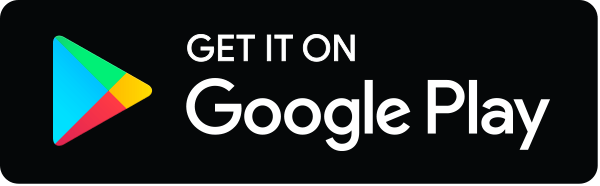