Fig. 20.1
This figure obtained from Cleveland Clinic Journal of Medicine with permission 2003 Oct; 70(10):866, 868, 871–873
The flow-volume loop is another aspect of data generated from spirometry characterized by a graphic illustration of inspiratory and expiratory flow (on the vertical axis) against volume (on the horizontal axis) during the performance of maximally forced inspiratory and expiratory maneuvers. Flow is plotted against volume to display a continuous loop from inspiration to expiration. The overall shape and the contour of the flow-volume loop are important in interpreting spirometric results (Fig. 20.2). In healthy subjects, maximal expiratory flow is highest at total lung capacity and progressively is reduced until residual volume is reached, while inspiratory flow is more even throughout the maximal effort of breathing in from residual volume to total lung capacity. In addition, the flow patterns are smooth during expiration or inspiration.


Fig. 20.2
Flow-volume loops
Obstructive Lung Disease: Another feature is the proportion of flow between inspiration and expiration, especially at mid-lung volumes, halfway between residual volume and total lung capacity. At this point, expiratory flow is less than inspiratory flow in those diseases in which there is intrathoracic airway disease, such as asthma, chronic obstructive pulmonary disease (COPD), or tracheomalacia. Patients with airflow limitation due to obstructive lung disease often have a concave upward pattern on the expiratory portion of the flow-volume loop.
Restrictive Lung Disease: The characteristic flow-volume loop pattern of restrictive disease is a decrease in vital capacity combined with supernormal expiratory flows when corrected for lung volume. The resulting shape of the flow-volume curve is a tall appearance with a steep descending limb.
Fixed Airway Obstruction: If flows are equal and reduced, there is a possibility of a fixed upper airway obstruction, such as tracheal damage from prior surgery or airway tumor/foreign body.
Variable extrathoracic obstruction: If inspiratory flow exceeds expiratory flow at mid-lung volume, this physiology is one of a variable extrathoracic obstruction, seen in people with tracheal injury, vocal cord paralysis, and other conditions where the act of breathing in restricts the size and flow of inspiration, while breathing out opens the segment that has dynamic collapse during inspiration (Fig. 20.3).


Fig. 20.3
Example of variable extrathoracic obstruction: note the “squaring” of the inspiratory limb of the flow-volume loop
Variable Intrathoracic Obstruction: Flow limitation is encountered during forced expiration, when the pleural pressure becomes positive relative to airway pressure, and the effect of any obstructive lesion becomes pronounced.
One feature of the loop that was thought to represent sleep apnea was a saw-tooth pattern on inspiration as well as on expiration believed to represent unstable upper airway structures (saw-tooth sign) [3] (Fig. 20.4). Although the precise mechanism may differ among individuals, fluttering on inspiration is not routinely seen in sleep apnea and can be present in those with motor disease like Parkinson’s disease and may also be seen in normal patients due to artifact [4]. Reversibility of airways obstruction can be assessed with the use of bronchodilators. After spirometry is completed, the patient is given an inhaled bronchodilator and the test is repeated. The purpose of this is to assess whether a patient’s pulmonary process is bronchodilator responsive by looking for improvement in the expired volumes and flow rates. In upper airway obstruction an increase in >12 % increase in the FEV1 (an absolute improvement in FEV1 of at least 200 ml) or the FVC after inhaling a beta agonist is considered a significant response. Spirometry is typically reported in both absolute values and as a predicted percentage of normal. Normal values vary depending on sex, race, age, and height.


Fig. 20.4
Saw-tooth pattern of flow-volume loop: this finding may be observed in the setting of an individual at increased risk of sleep apnea or a patient with neuromuscular disease. Alternatively, this finding also may simply reflect artifact
Lung Volumes
In contrast to spirometry, a dynamic test of airflow function, measurement of lung volumes is designed to collect values relating to the size of the lung as a gas-exchanging unit. These volumes are indirectly calculated from measures of a slow inspiration (or expiration) and a direct measure of thoracic gas volume (TGV) at functional residual capacity (FRC), with a subsequent derivation of a number of values. FRC is usually measured by a gas dilution technique or body plethysmography. Gas dilution techniques are based on a simple principle, are widely used, and provide a good measurement of all air in the lungs that communicates with the airways.
Body plethysmography is a second method of measuring lung volume that takes advantage of the principle of Boyle’s law, which states that the volume of gas at a constant temperature varies inversely with the pressure applied to it. The primary advantage of body plethysmography is that it can measure the total volume of air in the chest, including gas trapped in bullae. Another advantage is that this test can be performed quickly. Drawbacks include the complexity of the equipment as well as the need for a patient to sit in a small enclosed space. From the FRC, the patient pants against a closed shutter to produce changes in the box pressure proportionate to the volume of air in the chest. The volume measured by this technique is referred to as TGV and represents the lung volume at which the shutter was closed, typically FRC.
After measurement of FRC, the patient would exhale fully and then take a large breath to total lung capacity. The difference between FRC and residual volume is called the expiratory reserve volume (ERV), and the difference between the total lung capacity and FRC is called the inspiratory capacity. The ERV is reduced in obesity where the chest wall mass makes for a smaller FRC (Fig. 20.1).
The interpretation of lung volumes is coordinated with that of spirometry to allow one to distinguish between obstructive lung physiology and a mixed picture (Fig. 20.2). An obstructed pattern may be seen most commonly if there is narrowing of the airways due to bronchial smooth muscle contraction (i.e., asthma), narrowing of the airways due to inflammation and swelling of bronchial mucosa and the hypertrophy and hyperplasia of bronchial glands (i.e., bronchitis).
In some patients, obstructive sleep apnea may co-exist with COPD, which has been coined the “overlap syndrome” given that this is a clinically distinct entity compared with each disease in isolation [5]. Patients with the overlap syndrome have been shown to have a higher frequency of breathing and lower tidal volume compared with patients with sleep apnea [6]. In COPD, the oxygen saturation falls approximately 2–4 % from the awake baseline value with minor fluctuations until a more substantial reduction is noted in REM sleep, and therefore, nocturnal oxygen desaturation is a commonplace finding in the setting of obstructive lung disease (Fig. 20.5). A more pronounced degree of hypoxia occurs in the setting of COPD due to starting on a steeper portion of the oxyhemoglobin curve, alveolar hypoventilation due to reduction in chemosensitivity and reduction in end-expiratory lung volumes. The importance of the identification of overlap syndrome is highlighted by the observed association with increased risk of death and hospitalization because of COPD exacerbation [7]. Overlap syndrome has been associated with more pronounced sleep-related hypoxemia and hypercapnia compared with patients with COPD or OSA alone, which may explain the above findings [8]. The presence of OSA has also been demonstrated to result in increased COPD exacerbations and accelerated lung function decline [9].


Fig. 20.5
In a patient with both snoring and moderate COPD, the baseline is reduced during sleep but the saw-tooth pattern is suggestive of obstructive sleep apnea. A period of REM sleep
Restriction can be applied to patients whose total lung capacity has been measured and found to be significantly reduced. Total lung capacity is the volume of air in the lungs when the patient has taken a full inspiration. There are a variety of restrictive disorders such as intrinsic lung disease (sarcoidosis, interstitial lung disease), extrinsic restrictive lung disorders (morbid obesity, kyphosis/scoliosis), and neuromuscular restrictive disorders (myasthenia gravis, muscular dystrophy, post-polio syndrome, amyotrophic lateral sclerosis). Although preliminary findings appear to support reduced REM sleep and increased sleep fragmentation, overall there are limited data characterizing relationships of sleep and sleep apnea with restrictive parenchymal lung disease [10].
Measurement of maximal inspiratory and expiratory pressures is indicated whenever there is an unexplained decrease in vital capacity or respiratory muscle weakness is suspected clinically. Maximal inspiratory pressure (MIP) is the maximal pressure that can be produced by the patient trying to inhale through a blocked mouthpiece. Maximal expiratory pressure (MEP) is the maximal pressure measured during forced expiration (with cheeks bulging) through a blocked mouthpiece after a full inhalation. Repeated measurements of MIP and MEP are useful in following the course of patients with neuromuscular disorders.
Diffusing Capacity
Diffusing capacity measures the features at work involving movement of oxygen from the alveolar surface through to the hemoglobin molecule. The clinical test diffusing capacity of the lung (DL) most commonly uses carbon monoxide (CO) as the tracer gas for measurement because of its high affinity for binding to the hemoglobin molecule. This property allows a better measurement of pure diffusion, such that the movement of the CO is in essence only dependent on the properties of the diffusion barrier and the amount of hemoglobin. The properties of oxygen and its relatively lower affinity for hemoglobin compared with CO also make it more perfusion dependent; thus, cardiac output may influence actual measurement of oxygen diffusion measurements.
Diffusing capacity for CO (DLCO) is the measure of CO transfer. DLCO is a measure of the interaction of alveolar surface area, alveolar capillary perfusion, the physical properties of the alveolar capillary interface, capillary volume, hemoglobin concentration, and the reaction rate of CO and hemoglobin. The most widely used and standardized technique is referred to as the single-breath breath-holding technique. This technique relies on a subject inhaling a known volume of test gas, the patient inhales the test gas and holds his or her breath for 10 s and then exhales to “wash out” mechanical and anatomic dead space. DLCO is calculated from the total volume of the lung, breath-hold time, and the initial and final alveolar concentrations of CO. Alveolar volume is estimated by the helium dilution and the initial alveolar concentration of CO. The driving pressure is assumed to be the initial alveolar pressure of CO.
Because the level of hemoglobin present in the blood and diffusing capacity are directly related, a correction for anemic patients (DLCOc) is used to further delineate whether a DLCO is decreased due to anemia or due to parenchymal or interface limitation. Diseases such as interstitial pulmonary fibrosis or any interstitial lung disease may make the DLCO abnormal long before spirometry or volume abnormalities are present. Low DLCO is not only an abnormality of restrictive interstitial lung disease but may also occur in the presence of emphysema. On the other end of the spectrum, alveolar hemorrhage or congested capillary beds may actually increase the DLCO. Similar to spirometry, predicted formulas have been established for DLCO and DLCO corrected for alveolar volume. It is important to note, however, that differences in race have been observed in normal subjects, and a race correction of 7 % is allowed for African American patients [11]. The diffusing capacity, if reduced, may be a reason for oxygen levels to be low even when there are relatively normal values on spirometry or lung volumes. Such a circumstance is found with pulmonary vascular disease [12]. To the extent that a reduced diffusing capacity affects oxygen levels, it can be one reason for excess hypoxemia during sleep, even in the absence of apneas or hypopneas.
Arterial Blood Gas
A sample of arterial blood is drawn anaerobically from a peripheral artery (typically radial, however, brachial, axillary, or femoral routes may be considered) via a single percutaneous needle puncture, or from an indwelling arterial cannula or catheter for multiple samples. A resting arterial blood gas may be considered in the setting of hypoxia out of proportion to the degree of sleep-disordered breathing, thereby suggesting underlying possible cardiopulmonary disease or to obtain a sense of resting hypercapnia if underlying obesity hypoventilation, obstructive lung disease, or neuromuscular disease is known or suspected.
The arterial blood gas allows for the measurement of partial pressures of carbon dioxide (PaCO2) and oxygen (PaO2), acidity (pH), total hemoglobin (Hbtotal), oxyhemoglobin saturation (HbO2), carboxyhemoglobin (COHb), and methemoglobin (MetHb). Refer to Table 20.1 for the approach to acid–base determination. Arterial blood gases permit another assessment of gas exchange and of ventilation. One can derive a value of the A–a gradient to determine a problem in gas exchange at the alveolar level:
Table 20.1
Determination of acid–base status
Step I. Determine primary acid–base status disorder | |
(A) Determine acidosis versus alkalosis | |
1. pH < 7.36: Acidosis | 2. pH > 7.44: Alkalosis |
(B) Determine metabolic versus respiratory | |
1. Primary metabolic disorder (a) pH changes in same direction as bicarbonate, PaCO2 (b) Metabolic acidosis (i) Serum pH decreased (ii) Serum bicarbonate and PaCO2 decreased (c) Metabolic alkalosis (i) Serum pH increased (ii) Serum bicarbonate and PaCO2 increased | 2. Primary respiratory disorder (a) pH changes in the opposite direction of bicarbonate, PaCO2 (b) Respiratory acidosis (i) Serum pH decreased (ii) Serum bicarbonate and PaCO2 increased (c) Respiratory alkalosis (i) Serum pH increased (ii) Serum bicarbonate and PaCO2 decreased |
Step II. Determine compensation | |
(A) Metabolic acidosis: PaCO2 decreases 1.2 mmHg per each 1 meq/L bicarbonate fall | (A) Acute respiratory acidosis: Bicarbonate increases 1 meq/L per 10 mmHg PaCO2 rise |
(B) Metabolic alkalosis: PaCO2 increases 6 mmHg per 10 meq/L bicarbonate rise | (B) Chronic respiratory acidosis: Bicarbonate increases 4 meq/L per 10 mmHg PaCO2 rise |
(C) Acute respiratory alkalosis: Bicarbonate decreases 2 meq/L per 10 mmHg PaCO2 fall | |
(D) Chronic respiratory alkalosis: Bicarbonate decreases 4 meq/L per 10 mmHg PaCO2 fall |
![$$ {\text{P}}({\text{A}} {-} {\text{a}}){\text{O}}_{ 2} = [{\text{FiO}}_{ 2} \left( {{\text{P}}_{\text{B}} - {\text{P}}_{{{\text{H}}_{ 2} {\text{O}}}} ) - {\text{PaCO}}_{ 2} /{\text{RQ}}} \right] - {\text{PaO}}_{ 2} $$](https://i0.wp.com/neupsykey.com/wp-content/uploads/2017/10/A309636_4_En_20_Chapter_Equa.gif?w=960)
P(A–a)O2 = Alveolar-Arterial O2 Gradient. It is the difference between the amount of oxygen in the alveoli and the amount of oxygen dissolved in plasma.
FiO2 = Inspired Oxygen. It is the fraction of the inspired oxygen (0.21 at room air)
PB = Atmospheric Pressure of O2
= Partial Pressure of H2O
PaCO2 = Partial Pressure of CO2 in the Arterial Blood
PaO2 = Partial Pressure of O2 in the Arterial Blood
RQ: The Respiratory Quotient is the amount of CO2 relative to the amount of O2 consumed (in normal situations, this is approximately 0.8)
An increased A–a gradient would cause a reduction in arterial blood oxygen levels and be the result of a ventilation-perfusion mismatch (most common and most commonly observed in obstructive diseases such as COPD or asthma) or by an anatomic shunt (as in liver disease resulting in hepatopulmonary syndrome). A second pattern seen is a normal A–a gradient with the hypoxemia explained by hypoventilation of lung units, retention of carbon dioxide, and elevated arterial PaCO2. Hypoxemia due to pure hypoventilation (e.g., in obesity hypoventilation syndrome, neuromuscular disease resulting in CNS depression and impaired neural conduction) corrects with increase in fraction of inspired oxygen. A process resulting in hypoxemia during wakefulness is likely to produce lower oxygen saturation levels during sleep as the latter is associated with a reduction in the FRC and also reduced ventilation. Therefore, the presence of hypoxemia or hypoventilation in an awake resting arterial blood gas is predictive of greater hypoxemia during a sleep study.
Monitoring of Respiratory Function During Sleep
Updates have been put forth by the American Academy of Sleep Medicine (AASM) regarding scoring rules for respiratory events [13]. The goal of these updates was to revise the rules to reflect changes in our understanding of the predictiveness of various levels of hypoxia and flow reduction relative to adverse outcomes and also to address knowledge gaps in terms of specification of the sensor for detection of apnea and hypopnea during the positive airway pressure (PAP) titration given that PAP devices have the ability to output an analog or digital signal from the internal flow sensor. The updated rules also sought to overcome confusion caused by the endorsement of two hypopnea rules in the prior manual and to make more consistent the adult and pediatric respiratory event scoring rules. New evidence has emerged since 2007 showing the impact of the scoring rules in diagnosis of OSA [14, 15]. Below represents a description of the sensors that may be used for respiratory physiologic monitoring and also an overview of definitions recommended to be used for the scoring of respiratory events.
Airflow Monitoring
Most airflow sensors detect apneas reliably, but the detection and quantification of decreased flow needed to diagnose hypopneas depends on the type of sensor used. Hypopnea comprises the majority of obstructive respiratory events and therefore its measurement needs to be reliable.
The 2013 task force recommends thermistors, thermocouples, or polyvinylidene fluoride (PVDF) sensors for the detection of airflow. PVDF sensors have been included in the definition of thermal sensors for the detection of airflow given data demonstrating that the PVDF sum signal provides comparable detection of events independent of direct airflow monitoring with thermistry or nasal pressure. The PVDF sensor also performed as well as the pneumotachograph in the detection of the number of respiratory events [16, 17]. For identification of an apnea during the diagnostic study, the oronasal thermal airflow sensor is recommended to monitor airflow. If the oronasal sensor is not functioning or the signal is not reliable, then the use of nasal pressure transducer, respiratory inductance plethysmography (RIP) flow, or RIP sum is suggested. The nasal pressure signal is not the recommended sensor for apnea detection as the signal can show decreased amplitude during mouth breathing [18].
Pneumotachometer
This method of airflow monitoring provides a direct quantitative measurement of airflow or tidal volume and, however, requires connection to a sealed mask placed over the nose or mouth. It is considered the reference standard for obstructive apnea and hypopnea detection. Although its use provides a beneficial and accurate research tool, in the clinical setting this technique is somewhat cumbersome and therefore not standardly utilized. This method measures the flow rate of gases during breathing. The breath is passed through a short tube in which there is a fine mesh, which presents a small resistance to the flow. Flow (V′) is derived from the pressure difference over a small, fixed resistance, offered by a fine metal mesh. The pressure drop across the resistance relates linearly to flow at relatively low flows, when the flow pattern is laminar. Accurate measurements are best performed when the flow pattern is laminar and flow is linearly related to pressure drop.
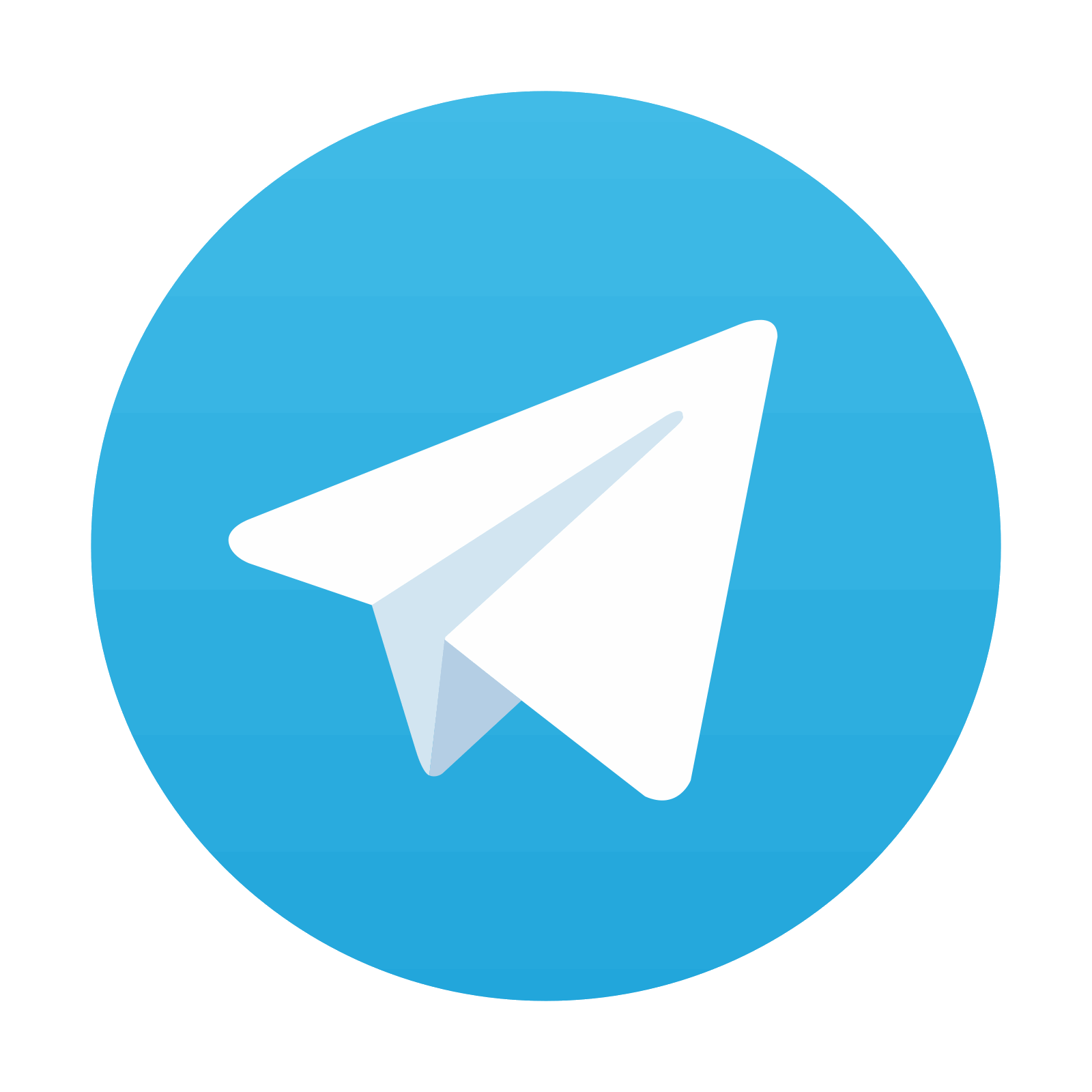
Stay updated, free articles. Join our Telegram channel
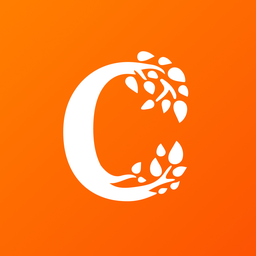
Full access? Get Clinical Tree
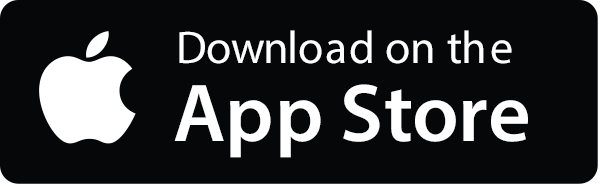
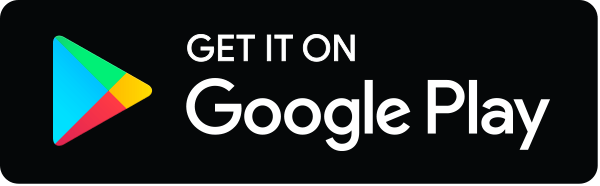