Multiple areas of the cortex are involved in motor movements. In addition to the cortex, there also exist multiple subcortical areas. During artificial simulation under anesthetics, the corticospinal fibers are the main carriers of that stimulation information to the alpha motor neurons in the spine. Even though the stimulation will activate fibers from other areas, this information is usually not passed due to the synaptic junctions between the other areas and the corticospinal tract which under anesthesia are shut down for the most part. (From http://thebrain.mcgill.ca/flash/a/a_06/a_06_cr/a_06_cr_mou/a_06_cr_mou.html (copy left) and with permission from the GNU free documentation license)
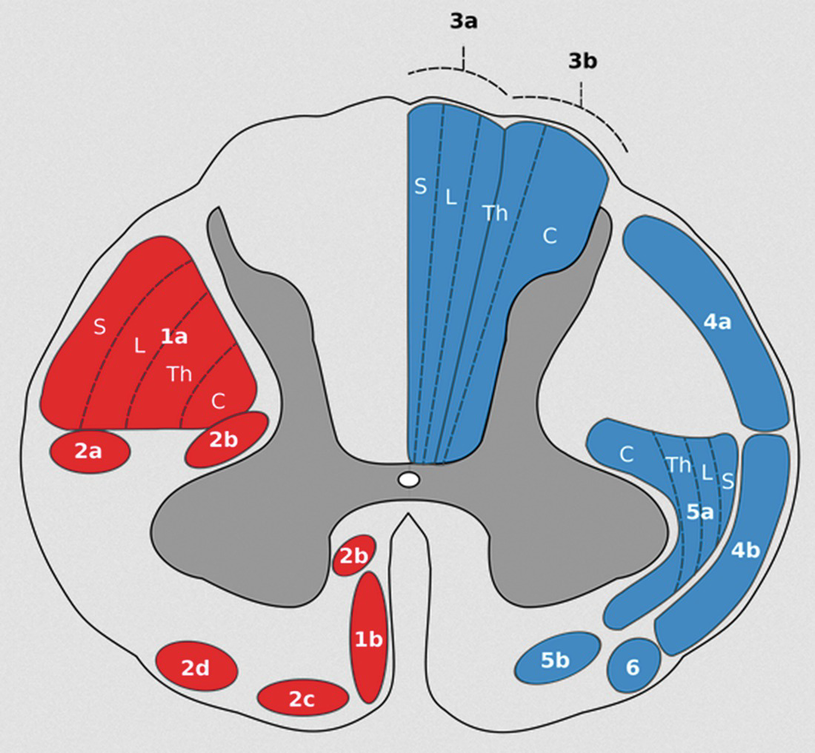
The lateral corticospinal tract (1a in the figure) shows a lateral to medial homunculus with the sacral region being most lateral and the cervical region being the most medial. Region 1b is the anterior corticospinal tract in the spinal cord. (With permission from Wikimedia Commons—public domain)
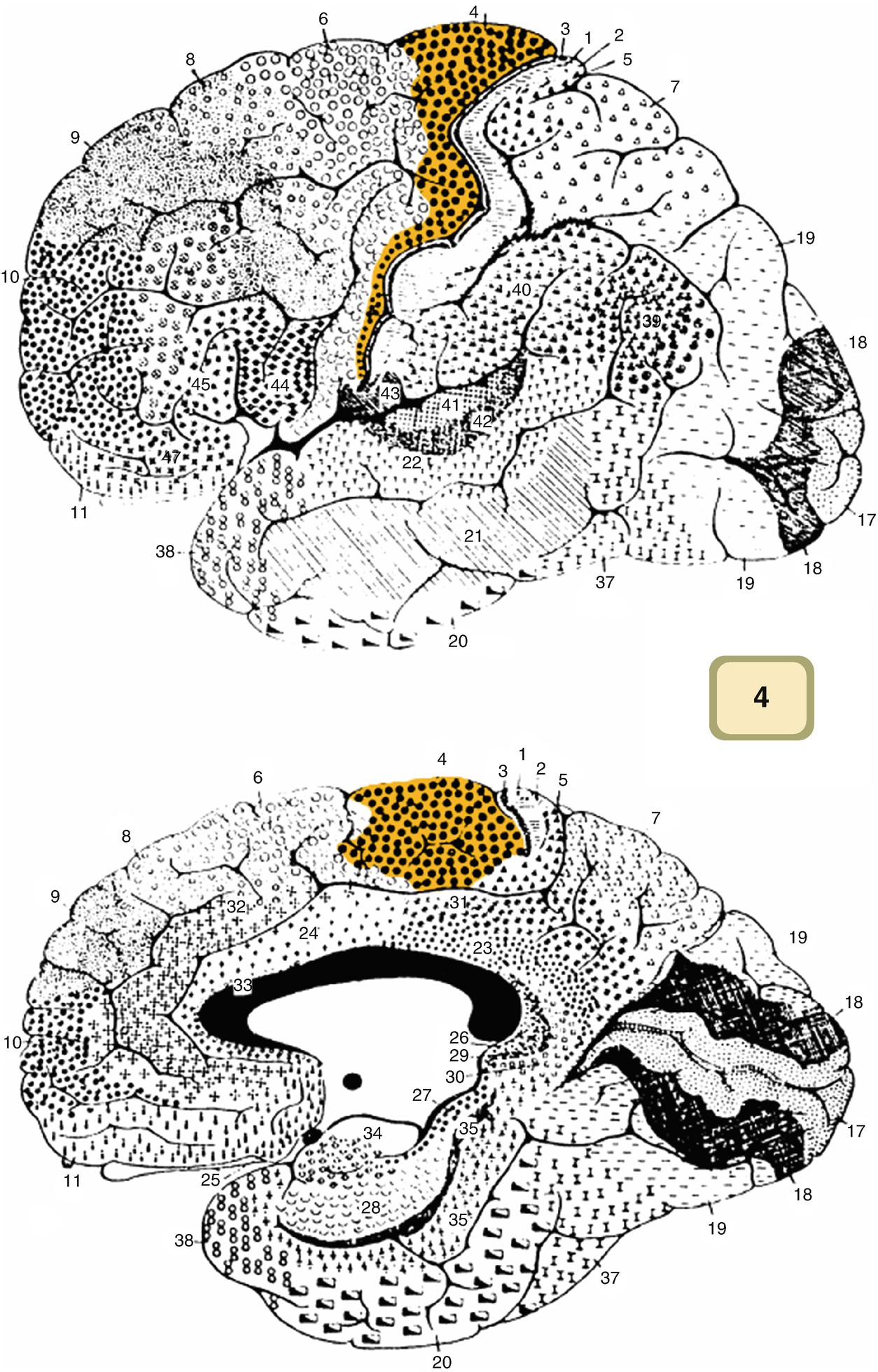
Map of the cortex with all of Brodmann areas depicted. Area 4, the primary motor cortex, is highlighted. Area 4 is just anterior to the central sulcus. (With permission from Wikimedia Commons—public domain)
There are about one million fibers in each CST with around 2% of these fibers being large (11–20 μm) which are known as fast-conducting corticospinal fibers (conduction around 50 m/s). CST fibers for the upper limb are more medial than lower limb fibers. The rest of the CST fibers synapse on other interneurons within the gray matter of the spinal cord. The large CST fibers are essential for eliciting MEPs. About 55% of all CST fibers end in the cervical region with 25% innervating the lower limbs. The rest of the fibers innervate the thoracic region. It is interesting to note that the CST is not symmetric, and it appears that CST fibers that cross more anterior tend to form the larger proportion of CST fibers in the cord whether it is the right or the left [25]. A single αMN has over 1000 synapses with over 50 direct inputs [26]; thus in the awake animal , generation of an action potential in the αMN is a complex process of competing systems. In the anesthetized animal, this complex system is shut down due to anesthetics. In addition to αMN CST inputs, there are inputs from interneurons driven by other CST fibers , inhibitory interneurons, Renshaw cells (which are inhibitory), sensory Ia and Ib fibers, and other descending tracts including the rubrospinal tract , vestibulospinal tract , reticulospinal tract , and tectospinal tract . Many of these presynaptic fibers synapse at multiple locations on the αMN, instead of one point. Due to the large number of synapses, it appears that the control of the αMN is multifactorial. In the non-neurologically compromised awake human, all the synaptic inputs to a specific αMN modulate the membrane potential; thus appropriate supratentorial modulation appropriately depolarizes the cell.
The CST enters the gray matter of the spinal cord in the ventral horn and fans out terminating in laminae IV through IX [27]. Yet the largest CST fibers appear to make monosynaptic connections to the αMN in laminae IX [28]. Most of the CST tends to synapse on interneurons, some of which being part of circuits that modulate the αMN, while others influence motor circuits such as the γ-motor system . Axons from the αMN innervate muscle fibers of a single muscle. The αMN and its axon are known as the lower motor neurons. The combination of the αMN, the terminal branches of the αMN, and the muscle fibers they innervate is known as the motor unit . Each motor unit is innervated by one axon and thus only one αMN. See Chap. 8 for more information on the motor unit.
Damage to either the upper motor neurons or the lower motor neurons will cause paralysis. Damage to the lower motor neuron will result in what is known as a flaccid paralysis —no muscle tone and no movement. Damage to the upper motor neuron demonstrates a more complex set of symptoms but generally includes no voluntary movement and a range of muscle tone from minimal tone to severe spasticity.
Indirect damage to the motor system can arise from reducing the blood supply to the critical structures. The cortex is supplied primarily by four main vessels, the two carotids and the two vertebral arteries. These four vessels supply the circle of Willis (COW) presenting connection between the carotid and vertebral arteries. The middle cerebral artery (MCA) coming off of the carotid artery supplies the lateral frontal and central cortex and its descending axons as well as much of the temporal lobes. The anterior cerebral artery (ACA) supplies the medial parasagittal frontal and central cortex and its descending axons originating from the motor cortex. Axons of the CST within the internal capsule are supplied by lenticulostriate branches originating from the MCA and the anterior choroidal arteries. At the level of the brainstem, the CST is supplied by branches of the vertebral and basilar arteries. The spinal cord is supplied by one anterior spinal artery (ASA) , two posterior spinal arteries (PSA) , and a varying number of radicular arteries. The ASA supplies the anterior 2/3 of the spinal cord including the lateral and anterior CST and the ventral horn. In the adult, the ASA is formed via fusion of the anterior spinal branches of the vertebral arteries, while the PSA originates from the posterior inferior cerebellar arteries (PICA) [29]. In the thoracic spinal cord, there is usually one large supply vessel coming from the aorta known as the artery of Adamkiewicz and two or three smaller vessels. Interestingly, in about 10% of patients, this vessel enters the spine at the L1–L2 level [30]. This variability in supply demonstrates one of the critical needs for neuromonitoring. Normally watershed zones are most commonly seen at levels T1, T5, and T8–T9 where reductions of blood flow in any of the feeder vessels can cause significant ischemia at these regions [29].
Electrophysiology
Electrical stimulation is used to generate APs in multiple points along the motor pathways. There are, in general, two types of recorded response in the anesthetized patient: (1) conducted volleys traveling along the spinal cord and peripheral nerves and (2) compound muscle action potentials (CMAPs) . The latter one records the muscle response activated from excitation of the αMN. In general stimulation is applied at the level of the motor cortex or subcortical part of the CST, the spinal cord, or peripheral nerve. Each of these areas requires different stimulation parameters that will be described in the next section.
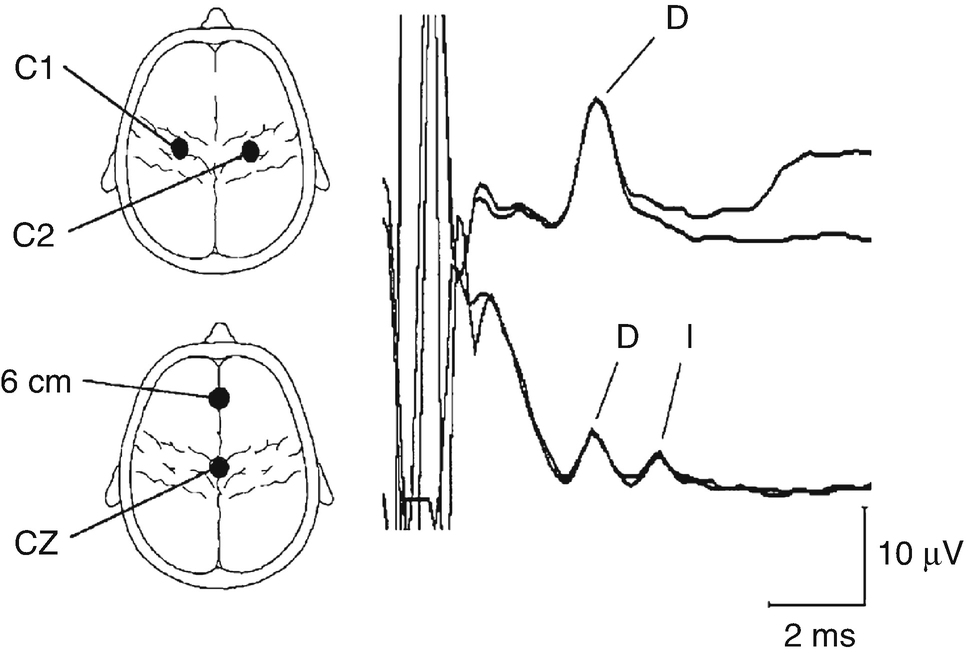
Upper thoracic epidural recordings of D- and I-waves in a 14-year-old female during surgery for a low cervical intramedullary tumor. The upper trace was obtained after transcranial electrical stimulation over C1 (anode) and C2 (cathode) using 140 mA stimulus intensity and a stimulus duration of 500 μs. The lower trace was obtained after anodic stimulation at Cz and cathodal stimulation at 6 cm anterior to Cz, using the same stimulus duration but at 200 mA. Note the appearance of the D- and I-waves with this electrode arrangement (An upward deflection is negative). (Reprinted with permission from Deletis [31])
Given that D-waves result from a direct activation of the cell body or axon, and the fact that the response is recorded from the axon, these responses are unaffected by anesthetics. I-waves, on the other hand, will usually not be present during certain forms of anesthetics given that they are generated via circuit pathways and contain multiple synapses . For many surgical procedures, such as during spinal instrumentation procedures, recording descending volleys along the CST is considered an invasive procedure and is not used. During most surgical procedures, muscle responses (known as CMAPs), resulting from driving the αMN via the largest fibers in the CT, are the monitored waves. Given that the αMN is a highly modulated cell, the effects of anesthesia are important in understanding the behavior of the CMAP response. As anesthesia starts to shut down synaptic transmission, it becomes increasingly difficult for a single pulse on one CT fiber to be able to generate a CMAP (although in some cases high-intensity long pulse stimulus durations will generate a CMAP). In order to compensate for the effect of anesthesia, it was found that a multi-pulse technique was necessary. It is important to remember that the multi-pulse technique is needed for generation of a CMAP, but the D-wave can be generated with a single pulse. It is interesting to note that when under deeper anesthetic states, I-waves are lost, thus giving credence to the synaptic nature of I-waves. When looking at the different anesthetic agents, it has been demonstrated that inhalational anesthetics are the most effective in abolishing the muscle MEP response [34–37]. It should also be noted that the blocking effects of inhalational anesthetics are not linear at the αMN; thus the interstimulus interval (ISI) between train of stimuli will need to change as concentrations change [35, 36]. Sloan et al. demonstrated that for low to moderate doses of isoflurane and N2O, an ISI between 3 and 6 ms was optimal for producing CMAPs, yet for high concentrations only 1 ms ISI produced a recordable CMAP. Given that they studied using N2O alone and found no major difference between the concentration and ISI, they concluded that isoflurane was the primary cause of the reduced CMAP amplitudes. At our institution we find that shorter ISIs help elicit MEPS when higher doses of inhalational agents are used and make less of a difference with a pure TIVA regime. A common technique to minimize the anesthetic effect at the αMN is to use an infusion of opioids with propofol . Even though this technique can still affect the CMAP response, its effect is much smaller than that of inhalational agents. Scheufler et al. investigated varying doses of propofol (combined with a constant remifentanil infusion) with different ISIs and stimulus intensities and found that an ISI of 1 ms produced the largest MEP response for a given dose of propofol [38]. It is important to note that in some cases, for patient safety, a specific anesthetic may be needed that is not optimally compatible when eliciting MEPs , and it is critical that the IOM technologist and neurophysiologist have a good line of communication with the anesthesiologist and surgeon.
There are additional pulse parameters that can help overcome the anesthetic effect at the αMN. The multi-pulse technique consisting of a train of 5–9 pulses and an ISI of 1–4 ms is the primary method. Some groups [39] describe using a 500 μs pulse width, while some IONM equipment does not allow for stimulation pulse widths above 75 μs.1 Recently, Abalkail et al. investigated pulses with optimization via strength duration curve analysis and found that a pulse width of 200 μs is optimal when using a 4 ms ISI [40]. In 1993, Taniguchi et al. studied multiple stimulation parameters during craniotomies [41]. Using both cathodal and anodal monopolar stimulation, Taniguchi et al. looked at stimulation pulse width, train length, and ISI. The study found that an ISI of 2 ms was optimal (i.e., minimal stimulation intensity to obtain a maximum MEP response) yet varied with age, anesthetic regime, and functional integrity. It is important to note that they did not do a systematic strength duration analysis though. Using these results some groups have demonstrated that when a non-optimal MEP is obtained, one should try varying the ISI (Journee et al. personal communication). Varying the ISI is important since for the αMN to reach firing threshold, the temporal relationship between the D-wave volleys is critical. Deletis et al. found that short trains with an ISI of 4 ms were optimal in eliciting responses in the tibialis anterior muscle (TA) based on complete recovery of the D-wave amplitude [18]. It is important to note that Taniguchi et al. used a 200 μs pulse width, while Deletis et al. used a 500 μs pulse width which may affect the optimal ISI. Deletis et al. demonstrate that if the ISI is a harmonic of the regular I-wave intervals, it will require less stimuli to be able to generate I-waves and in turn a CMAP, even though this may not be easy to determine in the OR other than by trying differing pulse widths if the response is difficult to obtain [18]. Szelenyi et al. found that an ISI of 4 ms always produced MEPs at the lowest stimulation threshold, yet the difference between the different ISIs was not statistically significant [42].
Another technique used to improve the efficacy of the CMAP is to use a conditioning pulse train [43]. The motor response recorded in the operating room is a combination of responses from multiple motor neuron pools. Each pool is directly activated by a single corticospinal axon. If all motor neuron pools are activated simultaneously, it would be easy to just modify the number of pulses in a train in order to elicit the maximum MEP amplitude . In most cases the motor neuron pools do not activate simultaneously when either giving a single pulse or single train of pulses due to dispersion (i.e., uneven conduction along the different fibers) between the fibers, even at supramaximal stimulation while under anesthesia. This dispersion has the effect of increasing the time difference between pulses arriving at the motor neuron pool. When there is a lesion in the fiber pathway, this dispersion effect increases. Thus, in many cases during surgery, the optimal MEP amplitude is not met due to abnormalities in the spinal cord fibers’ conductivity or impaired spinal cord function . The purpose of the conditioning pulse train is to raise the αMN membrane excitability. This pre-pulse train (conditioning pulse train) facilitates the generation of the CMAP via the actual test pulse train by making it easier for the test pulse train to depolarize αMN. This technique is based on the the following two properties: (1) increaseing the membrane excitability (depolarizing the alpha motor neuron membrane) via direct activation of the corticospinal tract; and (2) via secondary neurons activated by temporal summation. In order to optimize the facilitation, the test stimuli need to be applied just when the αMN membrane is maximally depolarized from the conditioning train. Journée et al. developed such a methodology whereby a pre-train is applied prior to the test train to raise the excitability of the αMN [43].
It is known that the motor threshold of a muscle during a voluntary contraction is lower than when that muscle is at rest and that this difference is modulated by both cortical and spinal mechanisms [44]. These voluntary mechanisms used to reduce motor threshold cannot be used when the patient is anesthetized. By using homonymous conditioning (stimulating the same pool at the same site for both the conditioning and test pulse train), there is the potential for a large overlap between the motor pool stimulated with the conditioning pulse and the test pulse. Journée et al. describe two windows for facilitation : (1) with an intertrain interval (ITI) between 10 and 40 ms and (2) with an ITI >100 ms. It is recommend trying the shorter ITI first and then the longer ITI [43].
Transcranial Motor Evoked Potentials
TES and TMS are both used to activate the motor system and elicit MEPs. The two techniques differ in their location of action on the neuron. With electrical stimulation the electrical current flows from the anode to the cathode, and the predominant direction of flow is in the radial direction, while for magnetic stimulation, the magnetic field passes perpendicular to the plane of the coil which is placed tangential to the scalp (see Fig. 7.2). The electric field produced by TMS is perpendicular to the magnetic field and thus tangential to the cortex. Thus for each type of stimulation, the electric field is oriented 90° from each other. When the electric field is parallel to the neural element, activation is a function of distance and also changes in orientation (i.e., not exactly parallel) of that element with bends being the most likely sites of activation [45, 46]. The TES response is at a slightly shorter latency than the TMS response [47]. The latency difference is a function of the trans-synaptic nature of TMS activation versus the direct activation of CST fibers when TES is utilized. As described above, when TES is applied in the awake animal, there is both a direct response (D-wave) from direct activation of the CST axons and also I-waves from indirect synaptic activation of the CST axons. Differing orientations of the coil will generate a response at differing latencies with respect to the D-wave produced by TES [48].
In the 1830s, Michael Faraday found that when a pulse of current is passed through a coil of wire, a magnetic field is generated. If a secondary conductor is nearby (within the induced magnetic field), a current is induced in this conductor that is related to the rate of change of the magnetic field [46]. When stimulating the brain using TMS, a coil is placed over the subject’s head, and a brief pulse (usually around 100 μs) is passed through that coil generating a magnetic field that is large enough to pass through the subject’s skull inducing a current within the brain. It is critical to point out that it is not the magnetic field that is directly stimulating the neural elements, but the secondary currents in the neural elements via induction. TMS has been tried during some surgical procedures [49], yet from a practical point of view due to the trans-synaptic nature of CT neuron activation, and the overall size of the stimulating element, TMS is not a suitable tool.
Electrical Elicited MEPs
The most common technique to elicit MEPs in the OR is via electrical stimulation applied to the scalp and/or exposed cerebral cortex and then to record the CMAP from the muscles. Using this technique, the functional integrity of both the CST and CBT can be continually monitored. The stimulus is applied over the motor cortex and recorded from the muscle or directly over the spinal cord. The montage and polarity used to apply the stimulation dictate the focalized nature of the stimulus, the laterality, and the extent of the artifact. For transcranial stimulation the montage can be categorized into bilateral (interhemispheric and midline) or unilateral (intrahemispheric). Using the international 10–20 EEG system, the standard MEP-stimulating electrodes are placed over the motor strip, and these are approximated with electrodes at positions C1, C2, C3, C4, and Cz, while for midline stimulation, having the cathode 6 cm anterior to Cz is also one possibility especially when muscle motor twitches disturb surgery. It should be noted that MacDonald recommends placing the leads a little more anterior to the standard central 10–20 locations and designates these as “M” locations [50]. The most common montages are the interhemispheric C1/C2(C2/C1) and C3/C4(C4/C3) montages. Making either C1 or C3, the anode will preferentially stimulate the CST fibers originating from the left hemisphere, while making either C2 or C4, the anode will preferentially stimulate the CST fibers originating from the right hemisphere. The C3/C4(C4/C3) montage is able to elicit muscle responses in all four limbs but is preferential for monitoring upper limb MEPs, while the C1/C2(C2/C1) shows a preference for the lower limbs, yet once again is able to elicit responses in all four limbs. The C3 and C4 montages have demonstrated the muscle activations with the lowest motor threshold in all four limbs [42] which might make it appear to be the most optimal for most MEP monitoring. An alternative is C1/C2 or C2/C1. C3/C4 and C4/C3 montages are known to cause large movement artifact. Instead of a focal stimulation, the stimulus is spread over a much larger area, in turn potentially activating many more fibers. Starting with the C3(C4)/C4(C3) montage, due to it having the lowest motor threshold, is a good solution. Yet, it needs to be kept in mind that this montage has the potential of deeper current penetration, and thus in supratentorial surgeries, such as aneurysm surgery, the stimulation point may be caudal to the site of the surgery and therefore can miss a lesion to the CST. In this case using the C1(C2)/C2(C1) montage may be more appropriate. Generally, in brain surgeries, direct stimulation of the exposed cortex via strip electrode is the method of choice. There are also other more focal montages such as the unilateral intrahemispheric C3/Cz and C4/Cz or the midline Cz/6 cm anterior to Cz. The C3(C4)/Cz montage was shown to be appropriate for eliciting upper limb responses but was very poor in eliciting lower limb muscle responses. The C3(C4)/Cz montage is the method of choice when eliciting corticobulbar responses such as those recorded from the vocal muscles [51] or the facial muscles [52]. The focal montage is superior to that of the interhemispheric montages since direct stimulation of the facial nerve itself can occur with the larger spreading montage, without actually stimulating the CBT. This response may also give a false sense of security since the stimulation location may be distal to surgery and thus give false-negative results if the injury occurs proximal to the stimulation point. To exclude the possibility that the current spreads distally and directly activates cranial nerves, and not corticobulbar fibers, the use of single stimulus versus train stimuli is needed [51, 53]. Finally in a rare set of patients, using the midline montage of Cz/+6 cm to Cz may be beneficial for eliciting muscle responses from the lower limbs, yet the stimulus intensity needs to be high.
Stimulation intensity varies along with the MEP technique used. A theoretical calculation by MacDonald et al. showed that using pulse widths between 50 and 800 μs should allow for safe stimulation (below the level of damage to neural tissue) and that using a pulse width of around 200 μs is optimal for energy minimization based on the rheobase and chronaxie of the stimulated neural elements [40, 50]. It should be noted that each patient is somewhat different, and patient-specific physiology, disease state, and the patient’s own response to anesthetic will affect the optimal stimulus parameters although the above ranges are good starting points.
At present there is no generally accepted ISI or train length as a standard for eliciting MEPs. Increasing the overall number of pulses within the train can reduce the stimulation threshold. It is also known that in some patients under light anesthesia, MEPs recorded from the muscles may be elicited by using one or two pulses, but in general the use of five pulses appears to be a good starting point [31]. Yet the use of more pulses (6–9) [54] or less pulses (3–4) [55, 56] is reasonable. Dong et al. [53] reported using three pulses when eliciting CBT MEPs. ISI starting points are also variable with the starting point ranging from 1 ms up to 4 ms. Szelényi et al. showed that using an ISI of 4 ms can minimize limb MEP thresholds [42], although using ISIs of 1 and 2 ms has shown to be best for both upper limb and CBT MEPs [50, 53, 57]. It is also worth mentioning that using an ISI of 2 ms is recommended for eliciting CBT MEPs because of their rather short latencies.
In the authors’ experience, a pulse train of seven pulses with an ISI of 2 ms and a pulse width of 75 μs is a reasonable starting point for generating limb and CBT MEPs. Yet as discussed by MacDonald et al. [50], individual patient characteristics and anesthetic conditions may require altering of the parameters to get an optimal MEP response.
Direct Cortical Stimulation
In addition to transcranial stimulation for eliciting muscle MEPs, one can also stimulate the cortical surface [58–61] or subcortical space [62] directly. In order to help localize the motor strip, mapping the location of the SSEP phase reversal is recommended and described elsewhere in this book . For direct cortical stimulation (DCS), it is highly recommended to use a four- to eight-contact strip electrode placed over the specific region of interest . In cases where the motor strip is exposed, using a stimulation probe to localize the motor cortex is recommended since this will help guide the placement of strip electrode. In some cases, such as during aneurysm surgery, it may not be possible to directly test the cortex since the strip is usually placed under the skull due to that region not being included in the exposure. Thus for MCA aneurysm procedure , the strip would be placed over the lateral motor strip, while for ACA procedures, the strip is placed more medially. The cathode is placed at FPz (or as close as possible) with the anode (active, stimulating electrode) being one of the electrodes on the strip. Similar stimulation parameters to TceMEPs are used for DCS stimulation except that stimulation intensity should not exceed 25 mA [58]. In this study published by Szelenyi et al. [58], they were able to record MEPs from DCS in 84% of cases. Reasons for not being able to elicit MEPs with this method include seizure , brain swelling, premature aneurysm rupture, subdural scars, and patients with an aneurysm in the posterior circulations (it should be noted that in a small subsection of patients with anterior circulation aneurysms, they did not place electrodes). Dislodgement of the electrode is an issue, yet we have found that once the electrode is in place, and by securing the lead wire with a staple, dislodgement of electrode was not an issue. One of the most frequent problems is the fact that the electrode contacts may not be over the motor strip . In some cases the surgeon might try to reposition the electrode, while in other cases, this has not occurred and we were not able to elicit MEPs. Szelenyi et al. have recommended that the surgeon uses the electrodes on the scalp when the exposure does not include the motor strip —the same ones used for TceMEPs—as a guide for placing the strip [58].
Once the electrode is placed, we start testing using a stimulation intensity of 10 mA if extradural or 3 mA if intradural and slowly increasing stimulation by 1–5 mA after five trials separated by 0.5–1 s. If no MEP response appears up to 25 mA for an extradural placement or 10 mA for intradural, then we switch the stimulating anode to the next electrode. We continue this until all electrodes are tested. The electrode with the lowest threshold is the one that is chosen to be used during monitoring. If no response is noted, we let the surgeon know this. The surgeon will then either reposition the electrode strip or continue without DCS MEPs.
In addition to stimulating the surface of the cortex, subcortical structures can also be mapped [62]. The primary reason for mapping these subcortical structures is to determine how close the resection is to the internal capsule. For these cases a monopolar stimulation probe is used. Using a pulse width of 75–500 μs (note that in the United States at the time of publication, a 500 μs was not available on all IONM devices) and a train of five to seven pulses with an ISI of 4 ms, stimulation is applied through a small ball tip probe of 1–2 mm. Stimulation intensity was increased to a maximum of 22 mA or until a muscle response was noted [62]. As the resection approaches the internal capsule, the threshold for CMAP activation reduces. It has been reported in the literature [63, 64] that the response to distance is 1 mA ≈ 1 mm. Thus for every decrease in stimulation intensity by 1 mA, the resection edge is 1 mm closer to the internal capsule . For distances greater than 5 mm between the resection cavity edge and the internal capsule, this ratio is acceptable. Yet, as the resection cavity becomes less than 5 mm away, the distance to threshold values becomes more nonlinear eventually approaching an asymptote (i.e., a minimal stimulation current needed to generate a response even if the probe is directly on the nerve). Seidel et al. demonstrate this effect by showing that as the threshold decreases to less than 3 mA, there is a significantly greater number of patients with permanent postoperative neurologic deficits compared to when the threshold stimulation amplitude is greater than 3 mA [62].
MEPs Recorded from the Muscles
Standard MEP monitoring uses the application of a stimulus at the head and the recording of potentials either from the spinal cord or muscle(s). The stimulus is applied via electrodes placed on the scalp for transcranial stimulation, overlying the dura, directly on the surface of the brain, or in the subcortical space. For transcranial stimulation, the subject matter of this chapter, gold cup electrodes, needle electrodes, or “corkscrew”-shaped electrodes could be used . Presently needle electrodes are the most commonly used, yet historically corkscrew and gold cup electrodes were preferred. Modern needle electrodes are of low impedance (around 400 Ω) which Journée et al. demonstrated [65]. MEP threshold is linearly related to impedance above 460 Ω, while below that MEP thresholds are constant [65]. Both the standard gold disk and the older needle electrode impedances are 800 and 1200 Ω, respectively. These electrodes are applied using the standard international 10–20 EEG system.2 MacDonald et al. recommended placing the central stimulating electrode 1 cm in front of the standard 10–20 system placement of C1, C2, C3, and C4. This location better corresponds to the motor strip . The FPz electrode is at the standard 10–20 system location [57].
Common muscle-nerve root mappings
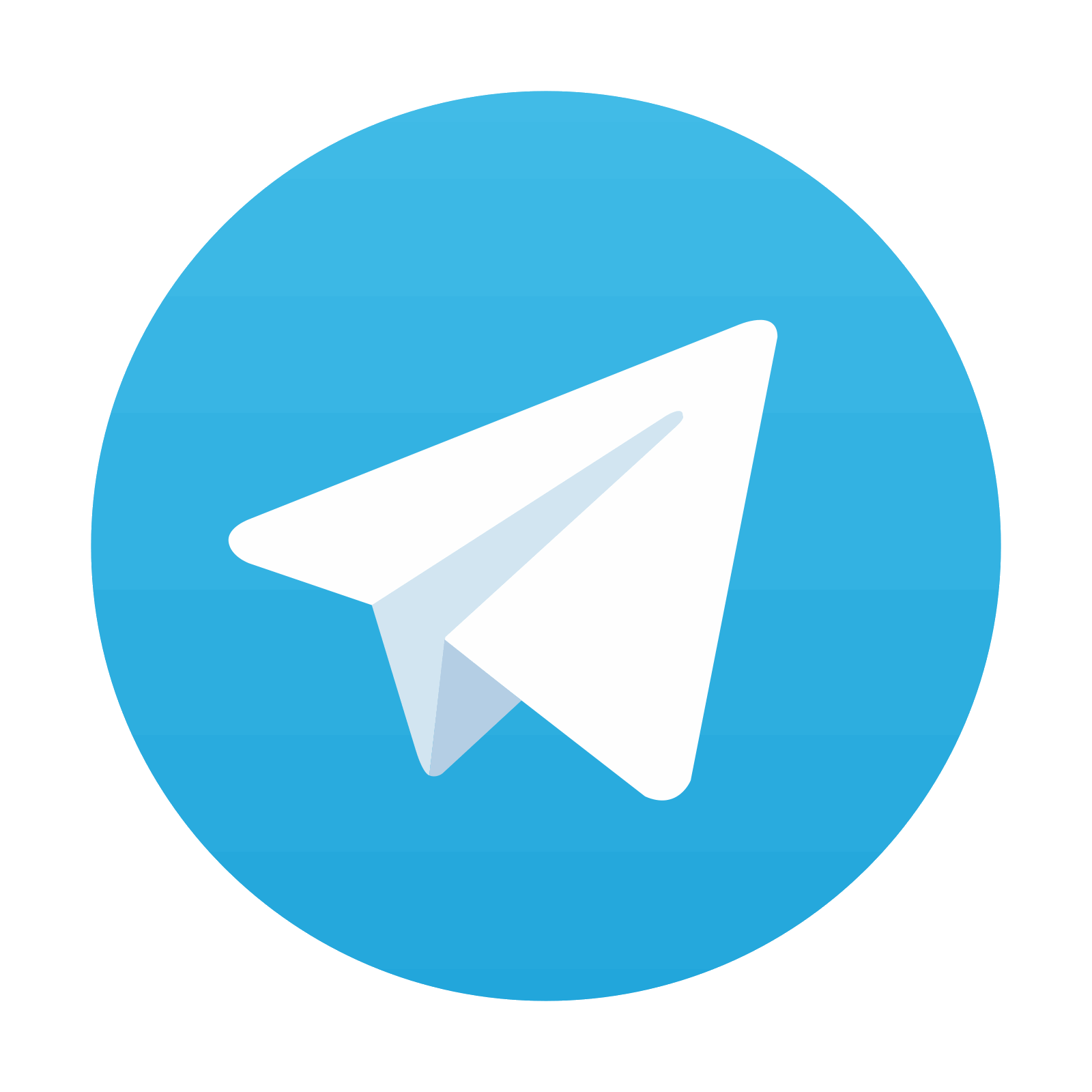
Stay updated, free articles. Join our Telegram channel
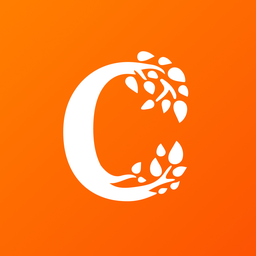
Full access? Get Clinical Tree
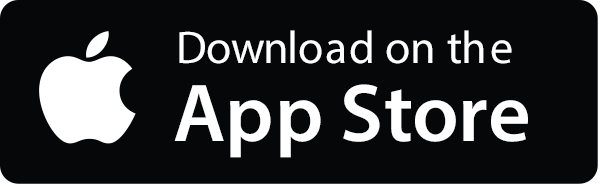
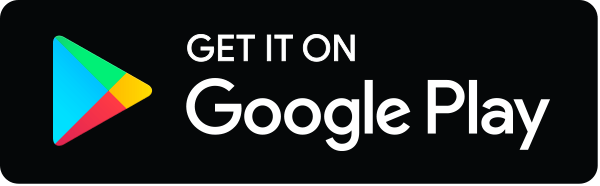