13 Surgical correction of scoliosis entails the risk of neurological injury. The risk varies with etiology, nature of the curve, and the type of procedure. For adolescent idiopathic scoliosis (AIS), the overall risk of neurological injury is under 0.5%; it is higher when accompanied by hyperkyphosis, a Cobb’s angle over 90°, or involves a combined anterior/posterior surgical procedure or revision surgery (1–3). Scoliosis of other etiologies, for example, congenital scoliosis and neuromuscular scoliosis also carry greater risks (4). Intraoperative neurophysiological monitoring (IONM) has been effective in reducing the risk of neurological injury during spinal deformity surgery by quickly detecting incipient injury and allowing the surgeon and anesthesiologist to take appropriate measures. The Therapeutics and Technology Assessment Subcommittee of the American Academy of Neurology and the American Clinical Neurophysiology Society have affirmed the ability of IONM, with somatosensory evoked potentials (SSEPs) and motor evoked potentials (MEPs), to effectively predict adverse neurological outcomes (5). Early on, Nuwer (6) demonstrated that monitoring of SSEPs during scoliosis surgery was accompanied by a nearly three-fold reduction in the rate of persistent severe postoperative neurological deficits. SSEP was initially proposed as a monitor of spinal cord function during spinal surgery in 1977 (7). By 1991, SSEPs were recognized as superior to the wake-up test for detection of spinal cord injury during scoliosis surgery (8); a series of 1,168 cases at Royal Orthopedic Hospital, Forbes (8), encountered no false-negatives and suggested that SSEP monitoring was more sensitive than, and should replace, the wake-up test. Nuwer’s 1995 multicenter study of over 51,000 monitored cases of scoliosis surgery confirmed the very low (0.063%) false-negative rate of SSEP monitoring. SSEPs are recorded by stimulating a peripheral nerve, usually the posterior tibial or peroneal nerve for lower extremity (LE) SSEPs and the median or ulnar nerve for upper extremity (UE) SSEPs, and recording over the scalp using electrode derivations designed to selectively record signals generated in the brainstem and in the primary sensory cortex. Cortical SSEPs are acquired using bipolar scalp-to-scalp derivations, whereas brainstem SSEPs are recorded using scalp-to-noncephalic derivations. It is helpful to record both brainstem and cortical SSEP signals because while each can serve as a monitor of dorsal column function, anesthetic agents and electrical interference in the operating room (OR) affect the two classes of signals differently. Cortical SSEPs are relatively resistant to muscle noise and electrical artifacts but can be suppressed by anesthetic agents. Brainstem SSEPs are much more susceptible to electrical noise and EMG artifact, but they are largely unaffected by anesthetic drugs. Brainstem SSEPs to UE and LE stimulation are quite similar (Figure 13.1), differing largely by latency, which reflects the length of the afferent pathway. SSEPs consist of a positivity, reflecting activity in the caudal medial lemniscus, followed by a longer-duration negativity (P14 and N18 for UE SSEPs, P31 and N34 for LE SSEPs) (16–18). Brainstem SSEP signals are conducted electrically through the brain and cerebrospinal fluid (CSF) to the scalp; they project over the entire scalp, and thus are easily recorded by any scalp electrode referred to any noncephalic electrode. In the OR, a cervical reference electrode is commonly used for recording brainstem SSEPs. The recorded signal is often called a “cervical” response, reflecting the common misconception that the cervical electrode is the principal “active” electrode in the scalp-cervical pair. While for UE SSEPs, an electrode over the lower cervical spine can add a postsynaptic cervical contribution (19), for LE SSEPs, the cervical electrode serves only as an “inactive’ noncephalic reference. FIGURE 13.1 Upper and lower extremity brainstem somatosensory evoked potentials, recorded from Fpz to a noncephalic reference. The responses are similar in appearance and consist of a positivity (P14 and P31) generated in the caudal medial lemniscus, followed by a negativity (N18 and N34), reflecting synaptic activity in the rostral brainstem. The scalp topography and polarity of the cortical components of UE and LE SSEPs differ, in part, because of the different locations of the hand and foot regions of the homunculus on the lateral hemispheric convexity and within the interhemispheric fissure, respectively. Cortical activation following median or ulnar nerve stimulation produces a negativity (N20) over the contralateral centroparietal scalp, overlying the hand area. UE SSEPs are recorded from an electrode on the contralateral centroparietal scalp (CPc) referred to a similarly positioned ipsilateral electrode (CPi). This arrangement allows for electronic removal of the underlying brainstem signals, detected by both electrodes, and recording of the cortical signal in isolation (16) (Figure 13.2). CPc-CPz may offer superior performance in the OR (20); the CPz reference provides for similar subtraction of underlying brainstem signals but with lower noise due to the shorter interelectrode distance. CPz-Fz is another commonly employed alternative. In this derivation, N20 signal amplitude may be enhanced by an approximately synchronous positivity often registered by the Fz electrode; in some patients, however, Fz may also introduce “noise” in the form of frontally maximal anesthetic-induced EEG activity (20). The situation for LE SSEPs is more complicated because the cortical location of the foot area varies considerably between people, from deep within the hemispheric fissure in some to near to the top of the fissure in others. In the former case, the LE cortical SSEP consists of a negativity (N38) over the contralateral hemisphere and a (approximately) simultaneous positivity (P38) over the ipsilateral hemisphere. In the latter situation, only a positivity (P38) is recorded at the vertex (21). LE SSEP scalp topographies of most individuals fall between these two extremes. For this reason, two or more channels are usually devoted to recording LE cortical SSEPs. For standard laboratory recordings, Cz-Fpz and Ci-Fpz are commonly used, selected to ensure detection of the P38 in each of its two extreme positions (Figure 13.3). In the OR, MacDonald observed that optimal recordings are often obtained using Cz-CPc or CPZ-CPc derivations. These derivations tend to produce high signal-to-noise ratio recordings because they employ short interelectrode distances, do not use a frontal reference, and often combine contributions from both the P38 and N38 signals (22). FIGURE 13.2 Left ulnar nerve somatosensory evoked potential (SSEP), recorded referential and bipolar derivations. The bipolar derivation (CP4-CP3) records the cortical SSEP in isolation, by electronically subtracting the brainstem potential (CP3-EPc) from the composite cortical plus brainstem potential (CP4-EPc). FIGURE 13.3 Two normal topographic variants of the P38 cortical somatosensory evoked potential (SSEP) following right tibial nerve stimulation. In variant A, a prominent positivity is recorded at the vertex, and very little is recorded over the lateral scalp; in variant B, a prominent positivity is recorded over the scalp ipsilateral to the stimulated limb and very little is recorded at the vertex. In the latter case, an approximately synchronous negativity (N38) is often recorded over the contralateral centroparietal scalp (not shown). The adequacy of peripheral nerve stimulation is confirmed by recordings of the afferent volley, from Erb’s point for UE SSEPs and in the popliteal fossa for tibial nerve LE SSEPs. While SSEPs can be recorded from the lumbar spine, reflecting postsynaptic activity in the lumbar enlargement of the spinal cord (N22) (23), and are important for outpatient diagnostic testing, they are not generally used for intraoperative monitoring. Both UE and LE SSEPs are usually monitored during scoliosis surgery. Since the region of spinal cord at risk is usually below the cervical cord, UE SSEPs function as “systemic” controls, that is, reflecting the effects of anesthesia, hypotension, or hypovolemia, rather than surgically related injury. On occasion, the cervical cord may be within the region at risk, for example, in patients with coexistent cervical cord abnormalities (24) or when orthopedic instrumentation extends to the cervical spine. Additionally, UE SSEPs are effective for detection of brachial plexus injury related to surgical positioning (25,26). Either surface disk electrodes or subdermal needle electrodes may be used for stimulation. For longer cases, needle electrodes tend to be more reliable; for patients with large circumference extremities, needle electrodes may be necessary for effective stimulation. For UE SSEPs, either median or ulnar nerve SSEPs may be recorded. Median SSEPs generally provide higher-amplitude, more robust signals for monitoring. Ulnar SSEPs can be sensitive to cervical cord injury below C6 that would go undetected by median SSEPs as well as to positioning-related brachial plexus stretch injuries. They are also useful for detecting intraoperative injury of the ulnar nerve at the elbow. For LE SSEPs, the tibial nerve is stimulated at the ankle. Alternatively, the common peroneal nerve (common fibular nerve) may be stimulated near the fibular head or in the popliteal fossa. The common peroneal nerve stimulation is technically more difficult and causes more patient movement than tibial nerve stimulation. Nonetheless, it can be a suitable alternative if the tibial nerve is difficult to stimulate. Also, it can be useful to record common peroneal SSEPs of patients in whom the popliteal fossa potential to tibial nerve stimulation is difficult to monitor; loss of scalp-recorded tibial SSEPs but preservation of peroneal SSEPs would imply a peripheral problem (eg, stimulation failure) rather than a spinal cord problem related to the surgery. Constant current simulators are preferred to constant voltage stimulators because of their ability to better compensate for variations in contact resistance. Stimulation rates of approximately 5 Hz are usually optimal (27), avoiding rates that are harmonically related to the power line frequency (60 Hz in North America). Higher rates may be used but they may produce lower-amplitude cortical responses. Lower rates usually offer no advantage and slow signal averaging; occasionally, however, particularly in patients with neurological abnormalities, rates between 1.5 and 3 Hz may improve responses. Stimulation intensity should be supramaximal. In healthy patients, stimulus intensities of 20 mA are often adequate; higher intensities, however, may be used as needed. Interleaved left/right stimulation may be employed, but simultaneous right and left stimulation should be avoided. Subdural needle electrodes are generally used for recording. They maintain good contact over lengthy operations, and, if dislodged, are easily replaced. Standard EEG disk electrodes may also be used and should be applied with collodion. Multichannel montages that include both cortical and brainstem channels should be used. It is important to include multiple cortical channels to account for known variations in signal scalp topography and to provide redundancy in case of electrode loss. Similarly, multiple brainstem channels can be helpful as they provide some redundancy for these typically “noisier” electrode derivations. It can be helpful to obtain recordings from a larger number of channels at baseline and then select a montage optimized to the patient’s scalp signal topography and to the noise environment (20,22). For UE SSEPs, a suggested montage is: CPc-CPi CPc-Fpz CPc-CPz Fpz-CS5 CPi-CS5 EPi-EPc For LE SSEPs, a suggested montage is: CPz-Fpz CPi-CPc CPz-CPc Fpz-CS5 CPi-CS5 PF1-PF2 Electrical interference, particularly at the frequency of power line alternating current (60 Hz in the North America), often presents a challenge to obtaining technically satisfactory recording in the OR. Equipment such as blood warmers, motorized patient tables, and operating microscopes are important sources of electrical noise. Care should be taken to run lead wires to avoid proximity to interference sources, when possible. It is often very helpful to twist or braid scalp-recording leads to maximize common mode rejection of power line interference. Band-pass filters of 30–1,000 Hz (-6 dB) are most commonly employed. However, most of the energy for SSEPs lies between 30 and 500 Hz, and high-frequency filters of 500 or even 300 Hz may be used effectively for monitoring, decreasing noise with only minimal effect of waveform morphology. Lower low-pass settings below 30 Hz offer no advantage and increase noise. Cortical SSEP signals are susceptible to attenuation by general anesthetic agents. Halogenated inhalations agents (eg, isoflurane, desflurane, sevoflurane) attenuate and also increase the latency of the N20 upper and P37 lower extremity SSEPs; nitrous oxide has similar effects (28–31) (Figure 13.4). The combined effect of nitrous oxide and halogenated agents appears to be greater than similarly potent doses of either drug alone (32). Infants and young children tend to be especially susceptible to these effects (33). Most intravenous agents (eg propofol, opioids, dexmedetomidine, benzodiazepines) have similar, but notably less prominent, effects (28,31,34). The degree of attenuation varies with not only the agent and the dose but also varies remarkably among patients (Figure 13.5). In some patients, ketamine and etomidate can be effective for enhancing SSEP cortical signals (35–37). Brainstem SSEPs signals (P14 and N18 for upper extremities, P31 and N34 for lower extremities), by contrast, are essentially unaffected by anesthetic agents in clinically relevant concentrations (34) (Figures 13.4 and 13.5). Brainstem SSEPs can be technically difficult to monitor, however, being more susceptible to contamination by both electrical noise and muscle artifact. The latter is a consequence of the long interelectrode distances required by scalp-to-noncephalic derivations as well as the location (often on the back of the neck) of the reference electrode. Neuromuscular blocking (NMB) agents can be used to eliminate EMG artifact and are sometimes necessary for monitoring brainstem SSEPs (Figure 13.6). Apart from technical issues, brainstem SSEPs may be difficult to record at baseline in patients with preexistent myelopathies. FIGURE 13.4 Effect of nitrous oxide on cortical lower extremity somatosensory evoked potentials (LE SSEPs). In a patient receiving balanced anesthesia with 70% nitrous oxide, 0.35% isoflurane, and propofol 35 ug/kg/min, the P38 cortical signal is barely detectable. When nitrous oxide is decreased to 0%, P38 quickly becomes dramatically larger. Holding nitrous oxide constant and decreasing isoflurane would have had a similar but slower effect, the speed reflecting isoflurane’s greater solubility in blood. FIGURE 13.5 Interpatient variability of the effect of anesthetic agents on cortical somatosensory evoked potentials (SSEPs). Patients A and B both received balanced anesthesia with 50% nitrous oxide, 0.5% isoflurane, and propofol 35 ug/kg/min. Cortical SSEPs are markedly suppressed in patient A but are sufficiently preserved in patient B to permit effective monitoring. Brainstem signals (arrows) are unaffected in both patients. Patient A received intermittent boluses of vecuronium to eliminate EMG artifact and permit monitoring of the brainstem SSEP. Either cortical or brainstem SSEPs can be sufficient for monitoring spinal cord function, as each serves as an indicator of dorsal column function. Their complementary strengths and weaknesses combine to enhance the reliability and flexibility of SSEP monitoring. Although some authors have recommended omitting brainstem SSEPs from routine SSEP monitoring protocols because they often have low signal-to-noise ratios and can be difficult to record (20), routine incorporation of brainstem channels in SSEP monitoring protocols adds flexibility and places fewer constraints on anesthetic choice. If cortical signals are robust, brainstem signals may be disregarded in favor of more rapid surgical feedback based on the cortical signals. If, however, for an individual patient and anesthetic protocol, brainstem responses are the most robust signals, then they should be used preferentially because they will, in fact, provide for the best and most rapid feedback to the surgeon. FIGURE 13.6 Use of neuromuscular blocking agents to facilitate monitoring based on brainstem somatosensory evoked potentials (SSEPs) in a 12-year-old with neuromuscular scoliosis and cerebral palsy, undergoing scoliosis surgery. Patient was receiving 0.25% isoflurane along with propofol 100 ug/kg/min. Cortical SSEPs are barely present and not monitorable. Following the administration of vecuronium, muscle artifact in the Fpz-CS5 channel is eliminated and the brainstem SSEP becomes monitorable (arrow). MEP monitoring during spinal surgery is performed by transcranial electrical stimulation of the motor cortex and recording the compound motor potential (CMAP) from muscle (M-wave) in the arms and legs. In direct spinal cord recordings, a single transcranial electrical stimulus can be seen to generate a series of efferent volleys: an initial single D (or direct)-wave followed over several milliseconds by a series of I (or indirect)-waves. D-waves result from direct activation of pyramidal cell axons. I-waves, in contrast, reflect firing of pyramidal cells in response to inputs from interneurons, themselves activated by transcranial electrical stimulation (38). Since no synapses are involved in D-wave generation, they are relatively resistant to anesthetic effects; I-waves, in contrast, are suppressed by most general anesthetics. Under general anesthesia, a single transcranial electrical stimulus is generally insufficient to fire spinal cord alpha motor neurons and elicit an M-wave. Instead, a rapid train of several transcranial electrical stimuli, typically 1 to several milliseconds apart, is required. The stimulus train elicits a corresponding train of D-waves plus some I-waves. The resultant synaptic inputs temporally summate, causing alpha motor neurons to reach threshold, fire, and produce the M-wave MEP (39) (Figure 13.7). FIGURE 13.7 Temporal summation by a spinal motor neuron. Each volley (corresponding to a D-wave or I-wave) causes further depolarization of the alpha motor neuron until it finally reaches threshold and fires, causing the innervated muscle fibers to fire. Recently, it has been shown that two sequential trains, separated by an appropriate intertrain interval (ITI), can often substantially enhance M-wave MEPs (Figure 13.8). The mechanism for this enhancement likely involves polysnaptic facilitatory circuits, both at the spinal and cortical levels. Proper timing of the trains is important because an incorrectly selected ITI can result in inhibition, rather than facilitation (40). Both UE and LE MEPs are usually monitored. As is the case with SSEPs, when the region of the spinal cord at risk is below the cervical cord, UE MEPs serve as “systemic” controls, reflecting the effects of anesthesia, neuromuscular blockade, hypotension, and hypovolemia. FIGURE 13.8 MEP facilitation by dual-train stimulation. Two stimulus trains are delivered, the second 20 milliseconds after the beginning of the first. Each train elicits an M-wave, the second much larger than the first. MEP stimulation may be performed using subdermal needle or corkscrew electrodes, or electroencephalogram (EEG)-type disk electrodes secured with collodion. Electrodes are most often placed at C1/C2 or C3/C4 scalp locations (41). C3/C4 locations can be more effective but also tend to elicit more face and jaw movement. Although MEPs are usually generated bilaterally, D-waves and hence M-waves are most reliably elicited by anodal stimulation (42). For monitoring, therefore, stimulator polarity is set so that the anode is contralateral to the recorded muscles; stimulator polarity is then switched when recording from the opposite side. Alternatively, the anode may be placed at Cz, with the cathode at Fz; with this arrangement, both legs may be effectively stimulated simultaneously, but UE muscle activation may be more difficult. In practice, it is useful to test several stimulating electrode placements and select the best one for the individual patient. M-waves are relatively large signals, often several hundred microvolts, so signal averaging is not needed. On the other hand, M-wave amplitude and morphology can vary from stimulation to stimulation, reflecting spontaneous fluctuation of the motor pool excitability. In some patients, this trial-to-trial variability of M-wave MEPs can be pronounced and can require several repeated stimuli to properly characterize (Figure 13.9). Either constant-voltage or constant stimulators can be employed; constant-voltage MEP stimulators are more common, largely for historic and regulatory reasons. Pulse train parameters should be optimized for the patient. In some patients, as few as three pulses may suffice; in others, six to eight may be needed. ITIs of 2 to 4 milliseconds are usually optimal (43–45). For dual-train stimulation, facilitation is greatest with ITIs of 10 to 20 milliseconds and again between 100 and 1,000 milliseconds (40). FIGURE 13.9 Variability of motor evoked potentials obtained sequentially under identical anesthetic and hemodynamic conditions. Anesthesia was achieved using propofol 125 ug/kg/min; mean arterial pressure was 60 mmHg. Abbreviation: RTA, right tibialis anterior. Subdermal or intramuscular needle electrodes, 2 to 3 cm apart, are used to record MEPs from the muscle belly. Belly tendon derivations can be used, but the longer interelectrode distance can be a source of added noise. For spinal cord monitoring, the most robust MEP signals are generally recorded from distal muscles controlled principally by the corticospinal tract. Abductor pollicis brevis and first dorsal interosseus for the upper extremity and adductor hallucis and tibialis anterior for the lower are commonly employed. Reliable MEPs can often be obtained from other muscles as well, and it can be helpful to include these in the selection of muscles for monitoring. For spinal cord monitoring, each muscle MEP serves as a surrogate for motor pathway integrity, probably because of the small size of the corticospinal tract and the likelihood that injury will affect many of its axons. Specific muscles, including proximal muscles and anal sphincter, can also be monitored for the purpose of detecting nerve root injury. While this can be effective, MEP nerve root monitoring is also more difficult and less reliable than MEP monitoring of spinal cord function. MEP monitoring of nerve root function is heavily dependent upon adequacy and stability of the particular muscle’s baseline response; its reliability is further constrained by the confounding effects of multisegmental innervation (46). Spinal cord D-waves are not monitored during scoliosis surgery because of the possibility of spurious amplitude changes resulting from movement of the epidural recording electrode with respect to the spinal cord during spinal deformity correction (47). Transcranial MEP monitoring is a safe procedure. Tongue bite and patient movement are the major risks. Twenty-five instances of tongue laceration were reported in a series of 18,862 MEP cases. Twenty-one were self-limited, requiring no intervention; only four required suturing (48). A soft bite block (eg, rolled gauze pads) should be used to prevent the tongue from coming in contact with the teeth. Patient movement is common with MEP stimulation, although it can often be minimized by appropriate adjustment of stimulation parameters (stimulation intensity, number of pulses). It is important to coordinate stimulation with the surgeon so that movement does not occur, for example, when surgical instruments are close to neural structures. No movement-related injuries were reported in a series of more than 18,000 cases involving MEP monitoring over 15 years (48). Epilepsy, shunts, cerebral lesions, skull defects close to stimulating electrodes, and implanted devices have been cited in the past as relative contraindications to transcranial electrical MEP (49). None, however, has been proven to result in complications related to MEP monitoring, and in many centers these do not presently preclude MEP monitoring (48,50). For individual patients, of course, the benefits of MEP monitoring need to be assessed, relative to potential risks. While D-waves are largely unaffected by commonly used anesthetics, these agents attenuate cortical I-waves (52) and also suppress spinal motor neurons (53). M-wave MEPs are particularly sensitive to both the halogenated inhalational agents and nitrous oxide (53,54). They are generally less severely affected by propofol and dexmedetomidine, and total intravenous anesthesia (TIVA), based on these agents combined with an opioid (eg, fentanyl, remifentanil) is commonly recommended for MEP monitoring (34,55). Nonetheless, modest doses of halogenated inhalational agents (generally < ½ minimum alveolar concentration [MAC]) or nitrous oxide can often be compatible with MEP monitoring (56–59). In our experience, dual-train MEP stimulation further facilitates flexibility in anesthetic selection (Figure 13.10). Low-dose ketamine can also be helpful, particularly in young children. While ketamine does not directly affect MEPs, it also does not inhibit I-waves. It can facilitate MEP monitoring by allowing lower doses of other anesthetics to be used (59,60). As with SSEPs, sensitivity to the suppressive effects of anesthetic agents varies considerably between patients. MEPs can be particularly sensitive to changes in anesthetic doses; boluses of anesthetic agents can transiently attenuate or even abolish responses. Even boluses of opioids, which in general only mildly affect MEPs (59), can also occasionally produce transient prominent attenuation. Stable anesthesia is ideal; when anesthetic adjustments are necessary, it is important that these be tracked and correlated with monitoring changes. Anesthetic changes that are likely to attenuate MEPs, and particularly their administration as boluses, are best avoided at times when close monitoring of MEPs is critical. NMB agents can be useful for improving SSEP signal quality, reducing patient movement, and perhaps reducing the incidence and severity of tongue bite injuries. If neuromuscular blockade is used, it must be partial, and the degree of blockade must be carefully monitored and titrated (48,59,61). The NMB agent should be given by a continuous infusion, because even small boluses tend to obliterate or substantially attenuate MEP signals (Figure 13.11). Most centers avoid the use of NMB agents in conjunction with MEP monitoring (59). In our experience, we have found that very low doses of vecuronium, titrated to three to four twitches on a “train of four” testing, can often notably improve SSEPs, particularly the brainstem signals, while not detracting from the quality of MEP recording (Figure 13.12). FIGURE 13.10 Dual-train stimulation can provide resilience to anesthetic effects on motor evoked potentials (MEPs). In a 12-year-old undergoing scoliosis surgery, anesthesia was provided using propofol 70 ug/kg/min and 0.3% isoflurane. Shortly after correction was achieved, 50% nitrous oxide was added, causing loss of the MEP response to the first train (thin arrow). Confusion, and a possible “false-positive” alert, were avoided by preservation of the response to the second stimulus train (thick arrow). Abbreviation: RAH, right abductor hallucis. SSEPs are routinely performed extraoperatively as part of the routine clinical evaluation of various neurological disorders. Extraoperative interpretation is based largely on interpeak latencies, reflecting conduction times between the various generators of the SSEPs signals. For UE SSEPs, these include EP-P14, P14-N20, and EP-N20, reflecting conduction times between Erb’s point and the lower brainstem, between the lower brainstem and cortex, as well as the overall conduction time between Erb’s point and cortex, respectively. For LE SSEPs, these include N22-P31, P31-P38, and N22-P38, reflecting, respectively, conduction times between lumbar spinal cord and lower brainstem, lower brainstem and cortex, and the overall conduction time between the lumbar cord and cortex. Interpretation is based on comparison of a patient’s latency values to normative data as well as differences between interpeak latencies measured following right- and left-sided stimulation. While the absence of expected signals also constitutes an abnormality, waveform amplitudes vary considerably among normal individuals and, as such, are generally not used in routine outpatient testing. FIGURE 13.11 Obliteration of motor evoked potentials (MEPs) by a small bolus of vecuronium. Forty minutes after receiving 6 mg of vecuronium for intubation, the patient had four of four twitches, and well-formed MEPs were obtained. The MEPs were then transiently abolished by a 1-mg bolus of vecuronium. Abbreviations: RAH, right abductor hallucis; RLG, right lateral gastrocnemius; RTA, right tibialis anterior. In the early days of intraoperative monitoring, it was common to obtain extraoperative SSEP recordings as a guide to what to expect in the OR. Although this practice continues to be advocated by some (62), it has been abandoned by many, in favor of simply relying on baselines obtained intraoperatively. This author generally favors the latter practice. In the absence of outpatient sedation, intraoperative recordings are usually of better technical quality; indeed, the brainstem P31 signal is frequently not recordable in unsedated outpatient recordings. Often, the most important information derived from baseline recording relates to the effect of anesthetics agents and can be assessed only in the OR. Preoperative assessment of MEPs, using transcranial magnetic stimulation, has been suggested (63). While this is feasible and could be useful in some cases, Food and Drug Administration (FDA)-approved devices are not currently available. In patients with AIS, SSEPs and MEPs are generally easy to obtain in the OR. While preoperative SSEPs could detect spinal cord abnormalities, such as Chiari malformations and syrinxes, that are occasionally present in patients with AIS (62), structural assessments are best made by using structural imaging techniques. That said, baseline latency-based SSEP abnormalities may be common in AIS, their reported prevalence varying from about 15% to nearly 100%. The abnormities appear to be subtle, however, and are best revealed using height-adjusted normative data and specifically evaluating interpeak intervals (62,64,65). Machida reported SSEP abnormalities in 97 of 100 AIS patients, finding the P31-P38 interpeak latency to be prolonged following stimulation of the tibial nerve ipsilateral to the concavity of the curve (65). This observation, which suggests a sensory system abnormality rostral to the lower brainstem, may provide an important clue to the pathogenesis of AIS. Indeed, disturbed somatosensory function and balance abnormalities have been described in AIS patients with abnormal SSEPs (64,66). FIGURE 13.12 Motor evoked potentials (MEPs) and somatosensory evoked potentials (SSEPs) recorded during surgery for adolescent idiopathic scoliosis (AIS) in a 12-year-old child receiving a vecuronium infusion at 1 mg/kg/hr, along with propofol and isoflurane. Train-of-four testing demonstrated three to four twitches. Robust MEPs were maintained, and with easily monitored brainstem SSEPs. Abbreviations: RAH, right abductor hallucis; RAPB, right abductor pollicis brevis; RTA, right tibialis anterior. For intraoperative monitoring, each patient’s responses are evaluated with respect to baseline signals, that is, the patient serves as his own control. This contrasts with standard laboratory testing, where patients’ responses are evaluated relative to normative population-based data. Baselines are obtained at the beginning of the case; it is often necessary to reestablish the baseline during the case, to account, for example, for changes in anesthesia. Even at constant anesthetic doses, both SSEPs and MEPs can change over time, a phenomenon known as “anesthetic fade.” Over hours, SSEP latencies are often seen to gradually increase and amplitudes to gradually decrease (67). Similarly, MEP threshold stimulation voltage commonly increases slowly over hours. The effect can be more striking in myelopathic patients (68). The mechanism for anesthetic fade is uncertain. It is seen both with lipid-soluble (eg, propofol), as well as lipid-insoluble (eg, N20) agents, and may be explained by slower time courses for some anesthetic effects, such as prolongation of refectory periods, than others (68). For SSEPs, the generally accepted “alarm criteria” are a 50% decrease in amplitude or a 10% increase in latency from baseline (69). Loss of SEP amplitude and degradation of signal morphology are the most common consequences of intraoperative spinal cord injury, which causes conduction block or desynchronization; latency changes are distinctly less prominent. In contrast to extraoperative testing, SSEP amplitude is therefore the most important parameter to monitor. It is a serious error to conclude that everything if okay simply because latencies have remained stable. The 50% amplitude 10% latency alarm criteria are empirically based and are best used as a guide, rather than a strict threshold above which it can be assumed that no adverse events have occurred. For one, noise can make it difficult to reliably distinguish a 60% from a 40% change from baseline. Further, it can be helpful to alert the surgeon to new, albeit smaller, SSEP changes that exceed their observed prior variability during the case. This approach can facilitate more accurate identification of the cause of SSEP changes and better affords the surgeon the option of acting immediately or taking a “wait and see” approach (70). There is no firm consensus on the alarm criteria for MEP monitoring. Suggested criteria include the presence or absence of a response, various percent loss of response amplitudes, decrease of M-wave complexity or duration, and increase in stimulus intensity required to elicit M-waves (71). Latency criteria are not useful (72). The complexity of MEP interpretation reflects, in part, the integrative role of the motor neurons in generating M-waves, requiring the temporal summation of descending volleys, as well as the trial-to-trial variability of MEPs. Complete loss of M-wave does not necessarily indicate complete loss of spinal cord motor function; indeed, M-waves can be lost when D-wave amplitude decreases by 30% to 50%, often resulting in a transient postoperative motor deficit (72). Although complete disappearance of the MEP has been a widely employed alarm criterion, once M-waves are lost, it is impossible to judge the degree of D-wave loss without direct spinal cord recording. It would seem, therefore, that a somewhat less strict alarm threshold is desirable to best ensure that the surgeon is notified before a possible “point of no return.” Given the commonly encountered degree of MEP variability, a 50% amplitude decrease criterion would likely cause too many false-positive notifications. The American Clinical Neurophysiology Society has suggested amplitude reduction of 75% to 90% as a reasonable threshold for alarm (41). The combined use of SSEPs and MEPs affords robust monitoring of spinal cord function during spinal deformity surgery. The two modalities rely on different signals, are mediated by different pathways, are monitored using different leads and cables, and may be differentially affected by anesthetic agents. Yet, both are usually affected by traumatic, compressive, and ischemic injury to the spinal cord. This redundancy can be critical to continued monitoring during real-life situations commonly encountered in the OR, which cause one or the other modality to fail or to become unreliable. If, for example, a bolus of propofol obliterates MEPs, monitoring could continue based on subcortical SSEP and perhaps cortical SSEP signals. If muscle or electrical artifact obscures the SSEPs, the much larger CMAP signals would likely permit continued MEP monitoring. Although, in the past, monitoring has focused on a “critical period” following correction, it is now clear that MEP and SEP changes reflecting incipient spinal cord injury can, and do, occur at any time during spinal deformity surgery. For this reason, monitoring should continue throughout the entire procedure, including during closure (73). Figure 13.13 illustrates a typical case of loss of SSEPs and MEPs during scoliosis surgery, with return of signals following modification of the correction. The patient is a 12-year-old girl undergoing posterior instrumentation for correction of AIS under total intravenous anesthesia, using propofol and fentanyl, plus partial neuromuscular blockade. Well-formed cortical (asterisk) and brainstem (arrow) SSEPs as well as MEPs are present at baseline (9:56, Figure 13A). By 16:05 (Figure 13B), correction has been achieved with placement of the first rod. As the second rod is placed, MEPs are lost on the left as well as in a single right LE muscle (VL) (arrows). SSEPs are unchanged. The surgeon is informed who begins removing the rods. For spinal cord monitoring, the response from each muscle serves as surrogate for right or left spinal cord motor function. Monitoring of responses from multiple muscles is useful, not only because of redundancy, in case one recording fails but also because responses from the muscles that are more difficult to stimulate (higher stimulation threshold voltage) can be lost first, and provide an “early warning” of possible further deterioration. Within several minutes (Figure 13C), MEPs are completely lost on both sides, while SSEPs remain unchanged. Only by 16:13 (Figure 13D), 8 minutes after the initial MEP loss, do SSEPs become attenuated bilaterally. MEPs often detect compromise of spinal cord function faster than SSEPs, in part because of the time required for SSEP signal averaging but also because they commonly prove to be more sensitive to spinal cord injury. By 16:21 (Figure 13E), with rods removed, MEPs have returned, and SSEPs are returning. Note that the first of the three superimposed SSEPs to be acquired (arrows) is of somewhat lower amplitude than the subsequent two recordings. At 16:43 (Figure 13F), the surgeon begins reinserting the rods, now shaped to achieve less aggressive correction of the scoliosis deformity. Six minutes later (Figure 13G), MEPs are again lost bilaterally; SSEPs remain unchanged. The surgeon is informed, the rods are released, and MEPs return (Figure 13H). Note that the first of the three superimposed SSEP recordings to be obtained does, in fact, show attenuation of the right brainstem (asterisk) and cortical (arrows) responses. FIGURE 13.13 A series of “screen shots” from a posterior instrumentation and fusion procedure for correction of scoliosis (see text for details). In each figure, three sets of superimposed tibial somatosensory evoked potentials (SSEPs) and two lower extremity motor evoked potentials (MEPs) are shown, with MEPs having been acquired following each of the first two sets of SSEPs. The labeled times correspond to the time of the first MEP. Right and left SSEPs were obtained simultaneously. VL, TA, and MG correspond to vastus lateralis, tibialis anterior, and medial gastrocnemius muscles, respectively. Ulnar SSEPs and abductor pollicis brevis MEPs as “systemic controls” are not shown, and they remained stable throughout the entire case. FIGURE 13.13 A series of “screen shots” from a posterior instrumentation and fusion procedure for correction of scoliosis (see text for details). In each figure, three sets of superimposed tibial somatosensory evoked potentials (SSEPs) and two lower extremity motor evoked potentials (MEPs) are shown, with MEPs having been acquired following each of the first two sets of SSEPs. The labeled times correspond to the time of the first MEP. Right and left SSEPs were obtained simultaneously. VL, TA, and MG correspond to vastus lateralis, tibialis anterior, and medial gastrocnemius muscles, respectively. Ulnar SSEPs and abductor pollicis brevis MEPs as “systemic controls” are not shown, and they remained stable throughout the entire case. FIGURE 13.13 A series of “screen shots” from a posterior instrumentation and fusion procedure for correction of scoliosis (see text for details). In each figure, three sets of superimposed tibial somatosensory evoked potentials (SSEPs) and two lower extremity motor evoked potentials (MEPs) are shown, with MEPs having been acquired following each of the first two sets of SSEPs. The labeled times correspond to the time of the first MEP. Right and left SSEPs were obtained simultaneously. VL, TA, and MG correspond to vastus lateralis, tibialis anterior, and medial gastrocnemius muscles, respectively. Ulnar SSEPs and abductor pollicis brevis MEPs as “systemic controls” are not shown, and they remained stable throughout the entire case. Rods are then reinserted, configured to achieve still less correction. This time, MEPs and SSEPs remain stable. Signals remain stable through closing (Figure 13I), and the patient awakens from anesthesia with intact spinal cord function. 1. Coe JD, Arlet V, Donaldson W, et al. Complications in spinal fusion for adolescent idiopathic scoliosis in the new millennium. A report of the Scoliosis Research Society Morbidity and Mortality Committee. Spine. 2006;31(3):345–349. 2. Diab M, Smith AR, Kuklo TR, et al. Neural complications in the surgical treatment of adolescent idiopathic scoliosis. Spine. 2007;32(24):2759–2763. 3. Qiu Y, Wang S, Wang B, et al. Incidence and risk factors of neurological deficits of surgical correction for scoliosis: analysis of 1373 cases at one Chinese institution. Spine. 2008;33(5):519–526. 4. Reames DL, Smith JS, Fu K-MG, et al. Complications in the surgical treatment of 19,360 cases of pediatric scoliosis. Spine. 2011;36(18):1484–1491. 6. Nuwer MR, Dawson EG, Carlson LG, et al. Somatosensory evoked potential spinal cord monitoring reduces neurologic deficits after scoliosis surgery: results of a large multicenter survey. Electroencephalogr Clin Neurophysiol. 1995;96(1):6–11. 7. Nash CL, Lorig RA, Schatzinger LA, et al. Spinal cord monitoring during operative treatment of the spine. Clin Orthop Relat Res. 1977;(126):100–105. 8. Forbes HJ, Allen PW, Waller CS, et al. Spinal cord monitoring in scoliosis surgery. J Bone Joint Surg Am. 1991;73-B:219–220. 9. Deecke L, Tator CH. Neurophysiol assessment of afferent and efferent conduction in the injured spinal cord of monkeys. J. Neurosurg. 1973;39:65–74. 10. Baskin DS, Simpson RK Jr. Corticomotor and somatosensory evoked potential evaluation of acute spinal cord injury in the rat. Neurosurgery. 1987;20(6):871–877. 11. Shiau JS, Zappulla RA, Nieves J. The effect of graded spinal cord injury on the extrapyramidal and pyramidal motor evoked potentials of the rat. Neurosurgery. 1992;30(1):76–84. 12. Machida M, Weinstein SL, Imamura Y, et al. Compound muscle action potentials and spinal evoked potentials in experimental spine maneuver. Spine. 1989;14(7):687–691. 13. Fehlings MG, Tator CH, Linden RD. The relationships among the severity of spinal cord injury, motor and somatosensory evoked potentials and spinal cord blood flow. Electroencephalography and Clincal Neurophysiology. 1989;74:241–259. 14. Ben-David B, Haller G, Taylor P. Anterior spinal fusion complicated by paraplegia. A case report of a false-negative somatosensory-evoked potential. Spine. 1987;12:536–539. 15. Merton PA, Morton HB. Stimulation of the cerebral cortex in the intact human subject. Nature. 1980;285(5762):227. 16. Mauguiere F, Desmedt JE. Bilateral somatosensory evoked potentials in four patients with long-standing surgical hemispherectomy. Ann Neurol. 1989;26(6):724–731. 17. Mauguiere F, Desmedt JE, Courjon J. Neural generators of N18 and P14 far-field somatosensory evoked potentials studied in patients with lesion of thalamus or thalamo-cortical radiations. Electroencephalogr Clin Neurophysiol. 1983;56(4):283–292. 18. Urasaki E, Tokimura T, Yasukouchi H, et al. P30 and N33 of posterior tibial nerve SSEPs are analogous to P14 and N18 of median nerve SSEPs. Electroencephalogr Clin Neurophysiol. 1993;88(6):525–529. 19. Emerson RG, Seyal M, Pedley TA. Somatosensory evoked potentials following median nerve stimulation. I. The cervical components. Brain. 1984;107(Pt 1):169–182. 20. MacDonald DB, Al-Zayed Z, Stigsby B, et al. Median somatosensory evoked potential intraoperative monitoring: recommendations based on signal-to-noise ratio analysis. Clin Neurophysiol, 2009;120(2):315–328. 21. Seyal M, Emerson RG, Pedley TA. Spinal and early scalp-recorded components of the somatosensory evoked potential following stimulation of the posterior tibial nerve. Electroencephalogr Clin Neurophysiol, 1983;55(3):320–330. 22. MacDonald DB, Al-Zayed Z, Stigsby B. Tibial somatosensory evoked potential intraoperative monitoring: recommendations based on signal to noise ratio analysis of popliteal fossa, optimized P37, standard P37, and P31 potentials. Clin Neurophysiol, 2005;116(8):1858–1869. 23. Emerson RG. Anatomic and physiologic bases of posterior tibial nerve somatosensory evoked potentials. Neurol Clin. 1988;6(4):735–749. 24. Maiocco B, Deeney VF, Coulon R, et al. Adolescent idiopathic scoliosis and the presence of spinal cord abnormalities. Preoperative magnetic resonance imaging analysis. Spine. 1997;22(21):2537–2541. 25. O’Brien MF, Lenke LG, Bridwell KH, et al. Evoked potential monitoring of the upper extremities during thoracic and lumbar spinal deformity surgery: a prospective study. J Spinal Disord. 1994;7(4):277–284. 26. Jones SC, Fernau R, Woeltjen BL. Use of somatosensory evoked potentials to detect peripheral ischemia and potential injury resulting from positioning of the surgical patient: case reports and discussion. Spine J. 2004;4(3):360–362. 28. Perlik SJ, VanEgeren R, Fisher MA. Somatosensory evoked potential surgical monitoring. Observation during combined isoflurane-nitrous oxide anesthesia. Spine. 1992;17(3):273–276. 29. Browning JL, Heizer ML, Baskin DS. Variations in corticomotor and somatosensory evoked potentials: effects of temperature, halothane anesthesia, and arterial partial pressure of CO2. Anesth Analg. 1992;74(5):643–648. 30. Sebel PS, Bowles SM, Saini V, et al. EEG bispectrum predicts movement during thiopental/isoflurane anesthesia. J Clin Monit. 1995;11(2):83–91. 31. Clapcich AJ, Emerson RG, Roye DP Jr, et al. The Effects of Propofol, Small-Dose Isoflurane, and Nitrous Oxide on Cortical Somatosensory Evoked Potential and Bispectral Index Monitoring in Adolescents Undergoing Spinal Fusion. Anesth Analg. 2004;99:1334–1340. 32. Sloan T, Sloan H, Rogers J. Nitrous oxide and isoflurane are synergistic with respect to amplitude and latency effects on sensory evoked potentials. J Clin Monit Comput. 2010;24(2):113–123. 33. Harper CM, Nelson KR. Intraoperative electrophysiological monitoring in children. J Clin Neurophysiol. 1992;9(3):342–356. 34. Sloan T, Heyer EJ. Anesthesia for intraoperative neurophysiologic monitoring of the spinal cord. J Clin Neurophysiol. 2002;19:430–443. 35. Agarwal R, Roitman KJ, Stokes M. Improvement of intraoperative somatosensory evoked potentials by ketamine. Paediatr Anaesth. 1998;8(3):263–266. 36. Schubert A, Licina MG, Lineberry PJ. The effect of ketamine on human somatosensory evoked potentials and its modification by nitrous oxide. Anesth. 1990;72(1):33–39. 37. Sloan TB, Ronai AK, Toleikis JR, et al. Improvement of intraoperative somatosensory evoked potentials by etomidate. Anesth Analg. 1988;67(6):582–585. 38. Amassian VE, Stewart M, Quirk GJ, et al. Physiological basis of motor effects of a transient stimulus to cerebral cortex. Neurosurgery. 1987;20(1):74–93. 39. Jones SJ, Harrison R, Koh KF, et al. Motor evoked potential monitoring during spinal surgery: response of distal limb muscles to transcranial cortical stimulation with pulse trains. Electroencephalogr and Clin Neurophysiol. 1996;100:375–383. 40. Journée HL, Polak HE, De Kleuver M. Conditioning stimulation techniques for enhancement of transcranially elicited evoked motor responses. Neurophysiol Clin. 2007;37(6):423–430. 41. American Clinical Neurophysiology Society. Guidleine on Transcranial Electrical Stimulation Motor Evoked Potentials (TES-MEP) Monitoring. ACNS. 0AD. http://www.acns.org/practice/guidelines. Accessed August 25, 2014. 42. Hern JE, Landgren S, Phillips CG, et al. Selective excitation of corticofugal neurones by surface-anodal stimulation of the baboon’s motor cortex. J Physiol. 1962;161:73–90. 43. MacDonald DB. Intraoperative Motor Evoked Potential Monitoring: Overview and Update. J Clin Monit Comput. 2006;20(5):347–377. 44. Deletis V, Isgum V, Amassian VE. Neurophysiological mechanisms underlying motor evoked potentials in anesthetized humans. Part 1. Recovery time of corticospinal tract direct waves elicited by pairs of transcranial electrical stimuli. Clin Neurophysiol. 2001;112(3):438–444. 45. Quinones-Hinojosa A, Lyon R, Zada G, et al. Changes in transcranial motor evoked potentials during intramedullary spinal cord tumour resection correlate with postoperative motor function. Neurosurgery. 2005;56:982–893. 46. Lyon R, Gibson A, Burch S, et al. Increases in voltage may produce false-negatives when using transcranial motor evoked potentials to detect an isolated nerve root injury. J Clin Monit Comput. 2010;24(6):441–448. 47. Ulkatan S, Neuwirth M, Bitan F, et al. Monitoring of scoliosis surgery with epidurally recorded motor evoked potentials (D wave) revealed false results. Clin Neurophysiol. 2006;117:2093–2101. 48. Schwartz DM, Sestokas AK, Dormans JP, et al. Transcranial electric motor evoked potential monitoring during spine surgery: is it safe? Spine. 2011;36(13):1046–1049. 49. . MacDonald DB. Safety of intraoperative transcranial electrical stimulation motor evoked potential monitoring. J Clin Neurophysiol. 2002;19(5):416–429. 51. Kobylarz EJ, Bilsky MH, Sandhu SK, et al. Monitoring of electroencephalography during transcranial electrical motor evoked potentials. Epilepsia. 2005;46(Suppl 8):309–310. 52. Burke D, Hicks R, Stephen J, et al. Assessment of corticospinal and somatosensory conduction simultaneously during scoliosis surgery. Electroencephalogr Clin Neurophysiol. 1992;85:388–396. 53. Zentner J, Albrecht T, Heuser D. Influence of halothane, enflurane, and isoflurane on motor evoked potentials. Neurosurgery. 1992;31(2):298–305. 54. Zentner J, Ebner A. Nitrous oxide suppresses the electromyographic response evoked by electrical stimulation of the motor cortex. Neurosurgery. 1989;24:60–62. 55. Bala E, Sessler DI, Nair DR, et al. Motor and somatosensory evoked potentials are well maintained in patients given dexmedetomidine during spine surgery. Anesthesiology. 2008;109(3):417–425. 56. Pelosi L, Stevenson M, Hobbs GJ, et al. Intraoperative motor evoked potentials to transcranial electrical stimulation during two anaesthetic regimens. Clin Neurophysiol. 2001;112(6):1076–1087. 57. Kempton LB, Nantau WE, Zaltz I. Successful monitoring of transcranial electrical motor evoked potentials with isoflurane and nitrous oxide in scoliosis surgeries. Spine. 2010;35(26):E1627– E1629. 58. Ubags LH, Kalkman CJ, Been HD. Influence of isoflurane on myogenic motor evoked potentials to single and multiple transcranial stimuli during nitrous oxide/opioid anesthesia. Neurosurgery. 1998;43(1):90–94. 59. Sloan T. Anesthesia and intraoperative neurophysiological monitoring in children. Childs Nerv Syst. 2010;26(2):227–235. 60. Frei FJ, Ryhult SE, Duitmann E, et al. Intraoperative monitoring of motor-evoked potentials in children undergoing spinal surgery. Spine. 2007;32(8):911–917. 61. Adams DC, Emerson RG, Heyer EJ, et al. Monitoring of intraoperative motor evoked potentials under conditions of controlled neuromuscular blockade. Anesth Analg. 1993;77(5):913–918. 62. Hausmann ON, Boni T, Pfirrmann CWA, et al. Preoperative radiological and electrophysiological evaluation in 100 adolescent idiopathic scoliosis patients. Eur Spine J. 2003;12(5):501–506. 63. Galloway GM, Brennan D, Brown JL. Transcranial magnetic stimulation—may be useful as a pre-operative screen of motor tract function. J Clin Neurophysiol. 2013;30(4):386–338. 64. Guo X, Chau WW, Hui-Chan CWY, et al. Balance control in adolescents with idiopathic scoliosis and disturbed somatosensory function. Spine. 2006;31(14):E437–E440. 65. Machida M, Dubousset J, Imamura Y, et al. Pathogenesis of idiopathic scoliosis: SEPs in chicken with experimentally induced scoliosis and in patients with idiopathic scoliosis. J Pediatr Orthop. 1994;14(3):329–335. 66. Loa MLM, Chow DHK, Guo X, et al. Impaired dynamic balance control in adolescents with idiopathic scoliosis and abnormal somatosensory evoked potentials. J Pediatr Orthop. 2008;28(8):846–849. 67. Kalkman CJ, Brink ten SA, Been HD, et al. Variability of somatosensory cortical evoked potentials during spinal surgery. Effects of anesthetic technique and high-pass digital filtering. Spine. 1991;16(8):924–929. 68. Lyon R, Feiner J, Lieberman JA. Progressive suppression of motor evoked potentials during general anesthesia: the phenomenon of “anesthetic fade”. J Neurosurg Anesthesiol. 2005;17(1):13–19. 69. American Clinical Neurophysiology Society. Guideline 11B: Recommended standards for intraoperative monitoring of somatosensory evoked potentials. ACNS. http://www.acns.org/pdf/guidelines/Guideline-11B.pdf. Accessed August 6, 2014. 70. Moller AR, Jho HD, Janetta PJ. Preservation of hearing in operations on acoustic tumors: an alternative to recording brainstem auditory potentials. Neurosurgery. 1994;34:688–692. 71. Langeloo DD, Journée HL, De Kleuver M, Grotenhuis JA. Criteria for transcranial electrical motor evoked potential monitoring during spinal deformity surgery. A review and discussion of the literature. Neurophysiol Clin. 2007;37:431–439. 72. Deletis V, Sala F. Intraoperative neurophysiological monitoring of the spinal cord during spinal cord and spine surgery: a review focus on the corticospinal tracts. Clin Neurophysiol. 2008;119(2):248–264. 73. Kamerlink RJ, Errico T, Xavier S, et al. Major intraoperative neurologic monitoring deficits in consecutive pediatric and adult spinal deformity patients at one institution. Spine. 2010;35:240–245.
Evoked Potentials in Adolescent Idiopathic Scoliosis: Intraoperative Neurophysiological Monitoring
SOMATOSENSORY EVOKED POTENTIALS
Physiological Basis
Brainstem Signals
Cortical Signals
Distal Monitors
Monitoring Setup
Stimulation
Recording
Anesthesia
MOTOR EVOKED POTENTIALS
Physiological Basis
Monitoring Setup
Stimulation
Recording
Safety
Anesthesia
INTERPRETATION
Preoperative Evaluation
Intraoperative Interpretation
Example Case
REFERENCES
Stay updated, free articles. Join our Telegram channel
Full access? Get Clinical Tree
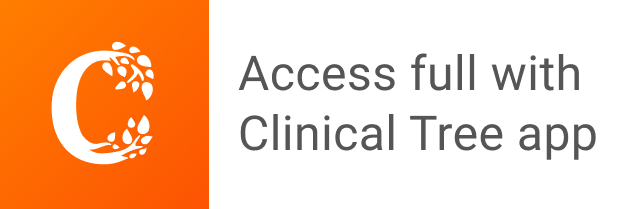