Experience and the Refinement of Synaptic Connections
Development of Human Mental Function Is Influenced by Early Experience
Development of Binocular Circuits in the Visual Cortex Depends on Postnatal Activity
Visual Experience Affects the Structure and Function of the Visual Cortex
Patterns of Electrical Activity Organize Binocular Circuits in the Visual Cortex
Reorganization Depends on a Change in the Balance of Excitatory and Inhibitory Inputs
Postsynaptic Structures Are Rearranged During the Critical Period
Synaptic Stabilization Contributes to Closing the Critical Period
Many Aspects of Visual System Development Are Activity-Dependent
Distinct Regions of the Brain Have Different Critical Periods of Development
Critical Periods Can Be Reopened in Adulthood
THE HUMAN NERVOUS SYSTEM IS FUNCTIONAL at birth: Newborn babies can see, hear, breathe, and suckle. However, the capabilities of human infants are quite rudimentary compared to those of other species. Wildebeest calves can stand and run within minutes of birth, and many birds can fly shortly after they hatch from their eggs. In contrast, a human baby cannot lift its head until it is 2 months old, cannot bring food to its mouth until it is 6 months old, and cannot survive without parental care for a decade.
What accounts for the delayed maturation of our motor, perceptual, and cognitive abilities? One main factor is that the embryonic connectivity of the nervous system, discussed in Chapters 52 through 55, is only a “rough draft” of the neural circuits that exist in our adult selves. After birth, embryonic circuits are refined by sensory stimulation—our experiences. This two-part sequence—genetically determined connectivity followed by experience-dependent reorganization—is a common feature of mammalian neural development, but in humans the second phase is especially prolonged.
At first glance this delay in human neural development might seem dysfunctional. Although it does exact a toll, it also provides an advantage. Because our mental abilities are shaped largely by experience, we gain the ability to custom fit our nervous systems to our individual bodies and unique environments. It could be argued that it is not the large size of the human brain but rather its experience-dependent maturation that makes our mental capabilities superior to those of other species.
The plasticity of the nervous system in response to experience endures throughout life. Nevertheless, periods of heightened susceptibility to modification, known as sensitive periods, occur at particular times in development. In some cases the adverse effects of deprivation or atypical experience early in life cannot easily be reversed by providing appropriate experience at a later age. Such necessary periods of development are referred to as critical periods. As we shall see, new discoveries are blurring the distinction between sensitive and critical periods, so we will use the term critical periods to refer to both.
Critical periods can most easily be appreciated from the perspective of behavior—the capacity to perceive the world around us, to learn a language, or to form strong social relationships. A 5-year-old child can quickly and effortlessly learn a second language, whereas a 15-year-old adolescent may become fluent but is likely to speak with an accent, even if he lives to be 90 years old. Likewise, when a cataract that deprives a child of vision is removed in early childhood, there are no lasting effects of the cataract, but when the surgery occurs at the age of 10 years, she is unlikely ever to have normal visual acuity. In each of these cases relevant experience must occur within a critical period if behavior is to develop normally.
The process of learning is also influenced by critical periods. One of the most striking illustrations of a lifelong behavior established during a critical period is imprinting, a form of learning in birds. Just after hatching, birds become indelibly attached, or imprinted, to a prominent moving object in their environment, typically their mother. The process of imprinting is important for the protection of the hatchling. Although the attachment is acquired rapidly and persists, imprinting can only occur during a critical period soon after hatching—in some species only a few hours. Thus postnatal development of the nervous system can be viewed as a series of critical periods.
We begin this chapter by examining the evidence that early experience shapes a range of human mental capacities, from our ability to make sense of what we see to our ability to engage in appropriate social interactions. To illustrate the neural basis of these experiential effects we describe the role of experience in the development of the visual system in experimental animals. Studies of the development of the visual system have provided our most detailed understanding of how experience shapes neural circuitry throughout the brain. We will see that experience is needed to refine patterns of synaptic connections and to stabilize these patterns once they have formed. Finally, we will consider recent evidence that critical periods may be less restrictive than once thought; in some cases they can be extended or “reopened”.
Understanding experience-dependent plasticity and the extent to which critical periods can be re-opened in adulthood has important practical consequences. First, much educational policy is based on the idea that early experience is crucial, so it is important to know exactly when a particular form of enrichment will be optimally beneficial. Second, medical treatment of many childhood conditions, such as congenital cataracts, is now predicated on the idea that early intervention is imperative if long-lasting deficits are to be avoided. Third, there is increasing concern that some behavioral disorders, such as autism, may be caused by impaired reorganization of neural circuits during critical periods. Finally, the possibility of reopening critical periods in adulthood is leading to new therapeutic approaches to neural insults such as stroke that previously were thought to have irreversible consequences.
Development of Human Mental Function Is Influenced by Early Experience
Early Experience Has Lifelong Effects on Social Behaviors
The conclusion that certain social or perceptual experiences are important for human development was first arrived at through studies of children who had been deprived of these experiences early in life. In rare cases children abandoned in the wild and later returned to human society have been studied. As might be expected, these children were socially maladjusted. Surprisingly, however, the defects proved to be generally irreversible.
In the 1940s the psychoanalyst René Spitz provided more systematic evidence that early interactions with other humans are essential for normal social development. Spitz compared the development of infants raised in a foundling home with the development of infants raised in a nursing home attached to a women’s prison. Both institutions were clean and both provided adequate food and medical care. The babies in the prison nursing home were all cared for by their mothers, who, although in prison and away from their families, tended to shower affection on their infants in the limited time allotted to them each day. In contrast, infants in the foundling home were cared for by nurses, each of whom was responsible for several babies. As a result, children in the foundling home had far less contact with other humans than did those in the prison’s nursing home.
The two institutions also differed in another respect. In the prison nursing home the cribs were open, so that the infants could readily watch other activities in the ward; they could see other babies play and observe the staff go about their business. In the foundling home the bars of the cribs were covered by sheets that prevented the infants from seeing outside. In reality, the babies in the foundling home were living under conditions of severe sensory and social deprivation.
Groups of newborn infants at the two institutions were followed through their early years. At the end of the first 4 months the infants in the foundling home fared better on several developmental tests than those in the prison nursing home, suggesting that intrinsic factors did not favor the infants in the prison nursing home. But by the end of the first year the motor and intellectual performance of the children in the foundling home had fallen far below that of children in the prison nursing home. Many of the children in the foundling home had developed a syndrome that Spitz called hospitalism and is now often called anaclitic depression. These children were withdrawn and displayed little curiosity or gaiety. Moreover, their defects extended beyond the emotional and cognitive. They were especially prone to infection, implying that the brain exerts complex controls over the immune system as well as behavior. By their second and third years, children in the prison nursing home were similar to children raised in normal families at home—they were agile, had a vocabulary of hundreds of words, and spoke in sentences. In contrast, the development of children in the foundling home was still further delayed—many were unable to walk or to speak more than a few words.
More recent studies of other similarly deprived children have confirmed these conclusions and shown that the defects are long-lasting. Longitudinal studies of orphans who were raised for several years in large impersonal institutions with little or no personal care, then adopted by caring families, have been especially revealing. Despite every effort of the adoptive parents, many of the children were never able to develop appropriate, caring relationships with family members or peers (Figure 56-1A). More recent imaging studies have revealed defects in brain structure correlated with, and presumably due to, this deprivation (Figure 56-1B).
Figure 56-1 Early social deprivation has a profound impact on later brain structure and behavior.
A. Neurocognitive dysfunction is evident in children raised under conditions of social deprivation in orphanages. The incidence of cognitive impairment increases with the duration of stay in the orphanage. (Reproduced, with permission, from Behen et al. 2008.)
B. Diffusion tensor magnetic resonance imaging (MRI) scans show a well-developed and robust uncinate fasciculus (red region) in a normal child (left), whereas in a socially deprived child (right) it is thin and poorly organized. (Reproduced, with permission, from Eluvathingal et al. 2006.)
C. Early social interactions impact later social behavior patterns. Monkeys reared in the presence of their siblings acquire social skills that permit effective interactions in later life (left). A monkey reared in isolation never acquires the capacity to interact with others, and remains secluded and isolated in later life (right). (Adapted, with permission, from Harry F. Harlow, University of Wisconsin.)
As compelling as these studies on humans are, it is difficult to derive definitive conclusions. An influential set of studies that extended the analysis of social behavior to monkeys was carried out in the 1960s by two psychologists, Harry and Margaret Harlow. The Harlows reared newborn monkeys in isolation for 6 to 12 months, depriving them of contact with their mothers, other monkeys, or people. At the end of this period the monkeys were physically healthy but behaviorally devastated. They crouched in a corner of their cage and rocked back and forth like autistic children (Figure 56-1C). They did not interact with other monkeys, nor did they fight, play, or show any sexual interest. Thus a 6-month period of social isolation during the first 18 months of life produced persistent and serious disturbances in behavior. By comparison, isolation of an older animal for a comparable period was found to be without such drastic consequences. These results confirmed, under controlled conditions, the critical influence of early experience on later behavior. For ethical reasons, these studies would not be possible today.
Development of Visual Perception Requires Visual Experience
The dramatic dependence of the brain on experience and the ability of that experience to shape perception is evident in people born with cataracts. Cataracts are opacities of the lens that interfere with the optics of the eye but not directly with the nervous system; they are easily removed surgically. In the 1930s it became apparent that patients who had congenital binocular cataracts removed after the age of 10 years had permanent deficits in visual acuity and had difficulties perceiving shape and form, a condition called amblyopia. In contrast, when cataracts that develop in adults are removed decades after they form, normal vision returns immediately.
Likewise, children with strabismus (crossed eyes) do not have normal depth perception (stereopsis), an ability that requires the two eyes to focus on the same location at the same time. They can acquire this ability if their eyes are aligned surgically during the first few years of life, but not if surgery occurs later in adolescence. As a result of these observations, congenital cataracts are now usually removed, and strabismus is corrected surgically, in early childhood. Over the past few decades researchers have elucidated structural and physiological underpinnings of these critical periods.
Development of Binocular Circuits in the Visual Cortex Depends on Postnatal Activity
Because sensory experience of the world is transformed into patterns of electrical activity in the brain, one might imagine that electrical signals in neural circuits affect the brain’s circuitry. But is this true? And if it is true, what changes occur and how does activity trigger them?
Our most detailed understanding of these links comes from studies of the neural circuits that mediate binocular vision. The key figures in the early phases of this work were David Hubel and Torsten Wiesel, who undertook a set of studies on cats and monkeys to investigate how experience affects the structural and functional organization they had delineated (Figure 56-2).
Visual Experience Affects the Structure and Function of the Visual Cortex
In one influential study Hubel and Wiesel raised a monkey from birth to 6 months of age with one eyelid sutured shut, thus depriving the animal of vision in that eye. When the sutures were removed it became clear that the animal was blind in the deprived eye. They then performed electrophysiological recordings from cells along the visual pathway to determine where the defect arose. They found that retinal ganglion cells in the deprived eye, as well as neurons in the lateral geniculate nucleus that receive input from the deprived eye, responded well to visual stimuli and had essentially normal receptive fields.
In contrast, cells in the visual cortex were fundamentally altered. In the cortex of normal animals most neurons are binocularly responsive. In animals that had been monocularly deprived for the first 6 months, most cortical neurons did not respond to signals from the deprived eye (Figure 56-3). The few cortical cells that were responsive were not sufficient for visual perception. Not only had the deprived eye lost its ability to drive most cortical neurons, but this loss was permanent and irreversible.
Figure 56-3 Responses of neurons in the primary visual cortex of a monkey to visual stimuli. (Adapted, with permission, from Hubel and Wiesel 1977.)
A. A diagonal bar of light is moved leftward across the visual field, traversing the receptive fields of a binocularly responsive cell in area 17 of visual cortex. Receptive fields measured through the right and left eye are drawn separately. The receptive fields of the two cells are similar in orientation, position, shape, and size, and respond to the same form of stimulus. Recordings (below) show that the cortical neuron responds more effectively to input from the ipsilateral eye. (F, fixation point.)
B. The responses of single cortical neurons in area 17 can be classified into seven groups. Neurons receiving input only from the contralateral eye (C) fall into group 1, whereas neurons that receive input only from the ipsilateral eye (I) fall into group 7. Other neurons receive inputs from both eyes, but the input from one eye may influence the neuron much more than the other (groups 2 and 6), or the differences may be slight (groups 3 and 5). Some neurons respond equally to input from both eyes (group 4). According to these criteria, the cortical neuron shown in part A falls into group 6.
C. Responsiveness of neurons in area 17 to stimulation of one or the other eye. The upper plot shows the responses of more than 1,000 neurons in area 17 in the left hemisphere of normal adult and juvenile monkeys. Neurons in layer IV that normally receive only monocular input have been excluded. The lower plot shows the responses of neurons in the left hemisphere of a monkey in which the contralateral (right) eye was closed from the age of 2 weeks to 18 months and then reopened. Most neurons respond only to stimulation of the ipsilateral eye.
Hubel and Wiesel went on to test the effects of visual deprivation imposed for shorter periods and at different ages. They obtained three types of results, depending on the timing and duration of the deprivation. First, monocular deprivation for a few weeks during the first 2 postnatal months led to loss of cortical responses from the deprived eye that was reversible after the eye had been opened, especially if the opposite eye was then closed to encourage use of the initially deprived eye. Second, monocular deprivation for a few weeks during the next months also resulted in a substantial loss of cortical responsiveness to signals from of the deprived eye, but in this case the effects were irreversible. Finally, deprivation in adults, even for periods of many months, had no effect on the responses of cortical cells to signals from the deprived eye or on visual perception. These results demonstrated that the cortical connections that control visual perception are established within a critical period of early development.
Are there structural correlates of these functional defects? To address this question we need to recall three basic facts about the anatomy of the visual cortex. First, inputs from the two eyes remain segregated in the lateral geniculate nucleus. Second, the geniculate inputs carrying information from the two eyes to the cortex terminate in alternating columns, termed ocular dominance columns. Third, lateral geniculate axons terminate on neurons in layer IVC of the primary visual cortex; convergence of input from the two eyes on a common target cell occurs at the next stage of the pathway, in cells above and below layer IVC (Figure 56-2).
To examine whether the architecture of ocular dominance columns depends on visual experience early in postnatal life, Hubel and Wiesel deprived newborn animals of vision in one eye and then injected a labeled amino acid into the normal eye. The injected label was incorporated into proteins in retinal ganglion cell bodies, transported along the retinal axons to the lateral geniculate nucleus, transferred to geniculate neurons, and then transported to the synaptic terminals of these axons in the primary visual cortex. After closure of one eye the columnar array of synaptic terminals relaying input from the deprived eye was reduced, whereas the columnar array of terminals relaying input from the normal eye was expanded (Figure 56-4). Thus sensory deprivation early in life alters the structure of the cerebral cortex.
Figure 56-4 Visual deprivation of one eye during a critical period of development reduces the width of the ocular dominance columns for that eye. (Scale bars = 1 mm.) (Adapted, with permission, from Hubel et al. 1977.)
A. A tangential section through area 17 of the right hemisphere of a normal adult monkey, 10 days after the right eye was injected with a radiolabeled amino acid. Radioactivity is localized in stripes (white areas) in layer IVC of the visual cortex, indicating areas of termination of the axons from the lateral geniculate nucleus that carry input from the injected eye. The alternating unlabeled (dark) stripes correspond to regions of termination of the axons carrying signals from the uninjected eye. Labeled and unlabeled stripes are of equal width.
B. A comparable section through the visual cortex of an 18-month-old monkey whose right eye had been surgically closed at 2 weeks of age. Label was injected into the left eye. The wider white stripes are the labeled terminals of afferent axons carrying signals from the open (left) eye; the narrow dark stripes are terminals of axons with input from the closed (right) eye.
C. A section comparable to that in part B from an 18-month-old animal whose right eye had been shut at 2 weeks. Label was injected into the right eye, giving rise to narrow white stripes of labeled axon terminals and wide dark stripes of unlabeled terminals.
How are these striking anatomical changes brought about? Does sensory deprivation alter ocular dominance columns after they have been established, or does it interfere with their formation? It is now clear that the mature pattern of ocular dominance columns in monkeys is not achieved until 6 weeks after birth (Figure 56-5). Only at this time do the terminals of fibers from the lateral geniculate nucleus become completely segregated in the cortex (Figure 56-6). Because the inputs are not well segregated at the time that visual deprivation exerts its effects, we can conclude that the deprivation perturbs the segregation of the inputs. We will see later that the remodeling of thalamic axonal arbors contributes to the perturbation of cortical columns.
Figure 56-6 The effects of eye closure on the formation of ocular dominance columns. The top diagrams show the gradual segregation of the terminals of lateral geniculate afferents in layer IVC of the visual cortex under normal conditions (left) and when one eye is deprived of stimulation (right). The blue domains represent the areas of termination of inputs from one eye, the red domains are those of the other eye. The lengths of the domains represent the density of the terminals at each point along layer IVC. For clarity the columns are shown here as one above the other, whereas in reality they are side by side in the cortex. During normal development layer IVC is gradually divided into alternating sites of input from each eye. The consequences of depriving sight in one eye depend on the timing of eye closure. Closure at birth leads to dominance by the open eye (red) because at this point little segregation has occurred. Closure at 2, 3, and 6 weeks has a progressively weaker effect on the formation of ocular dominance columns because the columns become more segregated with time. (Adapted, with permission, from Hubel, Wiesel, and LeVay 1977.)
Figure 56-5 The development of ocular dominance columns. Autoradiographs of four stages in the postnatal development of ocular dominance columns in the visual cortex in a cat. The images show horizontal sections through columns in the cortex ipsilateral to an eye that was injected with a radiolabeled amino acid. The cells in the lateral geniculate nucleus that receive input from the injected eye become labeled by transneuronal transport. At 15 days after birth the terminals of labeled fibers are spread in a relatively uniform manner along layer IV and are intermingled with those of unlabeled fibers that convey signals from the contralateral eye. At 3 and 5.5 weeks some segregation of the terminals is visible, but only as modest differences in labeling density. At 13 weeks the borders of the labeled bands become more sharply defined as the fibers conveying inputs from each eye segregate. (Adapted, with permission, from LeVay, Stryker, and Shatz 1978.)
Figure 56-2 Afferent pathways from the two eyes project to discrete columns of neurons in the visual cortex. Retinal ganglion neurons from each eye send axons to separate layers of the lateral geniculate nucleus. The axons of neurons in the lateral geniculate nucleus project to neurons in layer IVC of the primary visual cortex. Neurons in layer IVC are organized in alternating sets of ocular dominance columns; each column receives input from only one eye. The axons of the neurons in layer IVC project to neurons in adjacent columns as well as to neurons in the upper and lower layers of the same column. As a result, most neurons in the upper and lower layers of the cortex receive information from both eyes.
Patterns of Electrical Activity Organize Binocular Circuits in the Visual Cortex
What determines the extent of ocular dominance columns? The crucial factor may be the existence of minor differences in the proportion of inputs from each eye that converge on common target cells at birth. If by chance the fibers conveying input from one eye are initially more numerous in one local region of cortex, those axons may have an advantage.
How might this occur? An attractive idea, based on a theory first proposed in the 1940s by Donald Hebb, is that connections are strengthened when pre- and post-synaptic elements at a synapse are active together. In the case of binocular interactions, neighboring axons from the same eye tend to fire in synchrony because they are activated by the same visual stimulus at any instant. The synchronization of their firing means that they cooperate in the depolarization and excitation of a target cell. This cooperative action maintains the viability of those synaptic contacts at the expense of the noncooperating synapses.
Cooperative activity promotes branching of axons and thus creates the opportunity for the formation of additional synaptic connections with cells in the target region. At the same time, the strengthening of synaptic contacts made by the axons of one eye will impede the growth of synaptic inputs from the opposite eye. In this sense fibers from the two eyes may be said to compete for a target cell. Together, cooperation and competition between axons ensure that two populations of afferent fibers will eventually innervate distinct regions of the primary visual cortex with little local overlap.
Competition and cooperation are not simply the outcome of neural activity per se, or of differences in absolute levels of activity among axons. Instead, they appear to depend on precise temporal patterns of activity in the competing (or cooperating) axons. The principle was dramatically illustrated by Hubel and Wiesel in a set of studies that examined stereoscopic vision—the perception of depth. The brain normally computes depth perception by comparing the disparity in retinal images between the two eyes. When the eyes are improperly aligned, this comparison cannot be made and stereoscopy is impossible. Such mis-alignments occur in children who are “cross-eyed,” or strabismic. As noted, this condition can be surgically repaired, but unless the surgery occurs during the first few years of life, the children forever remain incapable of stereoscopy.
Hubel and Wiesel examined the impact of strabismus on the organization of the visual system in cats. To render cats strabismic, the tendon of an extraocular muscle was severed in kittens. Both eyes remained fully functional but misaligned. Inputs from the two eyes that converged on a binocular cell in the visual cortex now carried information about different stimuli in slightly different parts of the visual field. As a result, cortical cells became monocular, driven by input from one eye or the other but not both (Figure 56-7). This finding suggested to Hubel and Wiesel that disruption of the synchrony of inputs led to competition rather than cooperation, so that cortical cells came to be dominated by one eye, presumably the one that had dominated it very slightly at the outset.
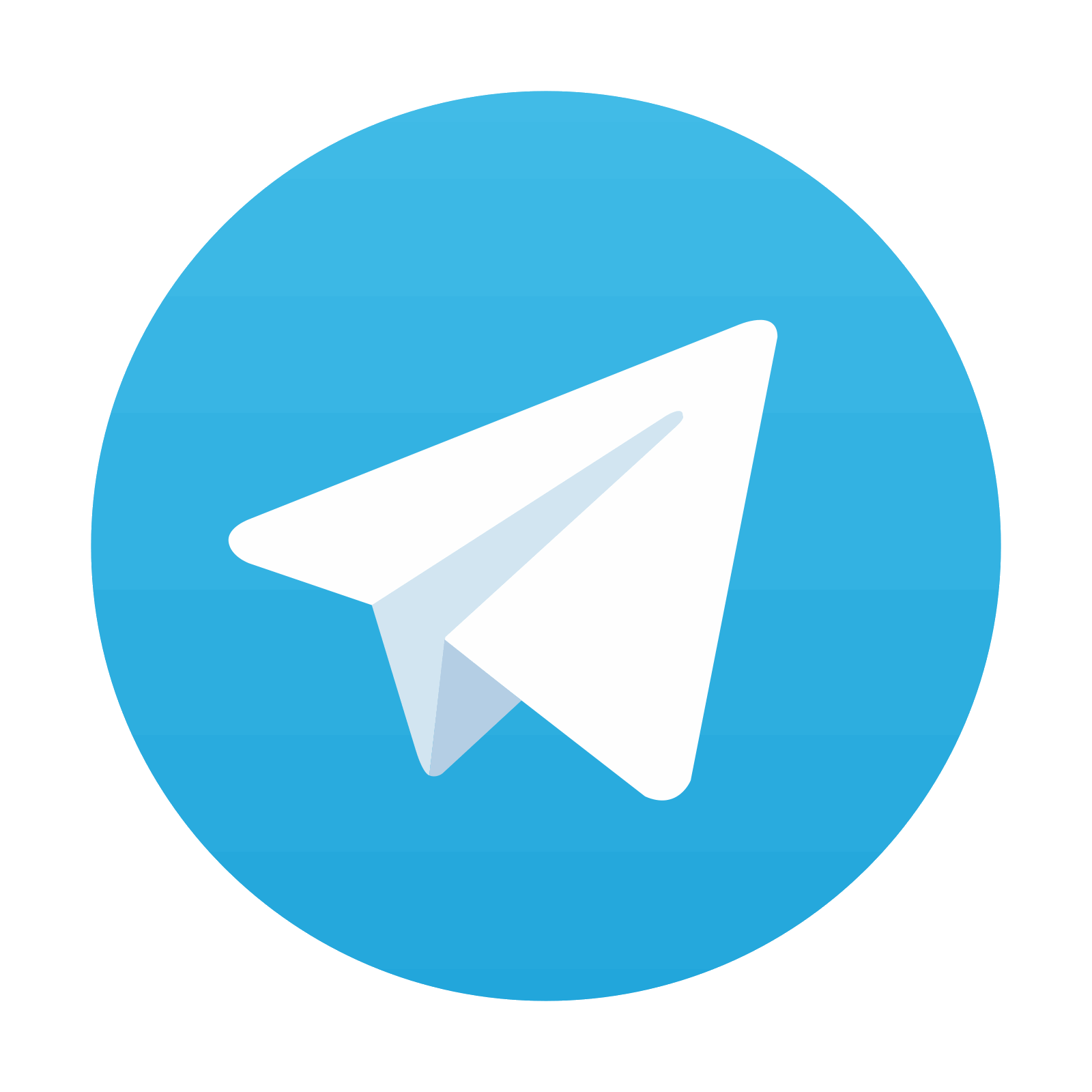
Stay updated, free articles. Join our Telegram channel
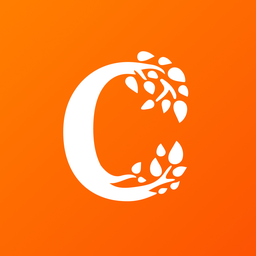
Full access? Get Clinical Tree
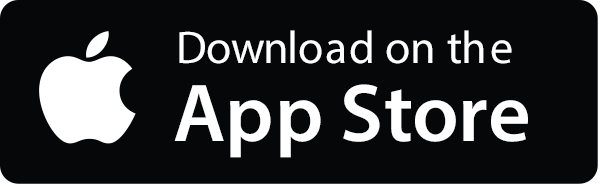
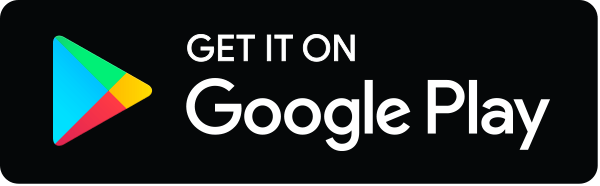