Extratemporal Epilepsy Surgery Surgical procedures for extratemporal lobe epilepsy (ETLE) have been built on the success of temporal lobe resections. In 1990, the National Institutes of Health adopted surgical resection as an alternative treatment to medication-resistant epilepsy in children.1 Temporal lobe resection, described in Chapter ▶ 72, has demonstrated significant success in reducing seizures in selected children.2 A randomized controlled trial for temporal lobe epilepsy revealed improved seizure freedom for patients with surgical intervention versus medical therapy.3 Although it might appear that outcomes after ETLE surgery could approximate those seen after temporal lobe resections, a wide range of pathologies, diagnostic evaluations, and surgical approaches contributes to substantial variability in the outcomes of ETLE surgery. Extratemporal pathologies account for a substantial majority of cases of ETLE in children, whereas in adults, temporal lobe epilepsy predominates. However, children treated for ETLE may also have temporal lobe involvement as part of an underlying multilobar pathology. Typically, these extratemporal areas are the frontal, occipital, and/or parietal lobes, and eloquent cortex in these regions may be affected. ETLE also presents an additional degree of difficulty in localization because seizures can quickly propagate to additional locations throughout the cortex. Extratemporal surgical approaches are employed to address a broad range of pathologies, from tumors to malformations of cortical development, vascular lesions, and other genetically inherited disorders.4 Each disease state has its own particular evolution, symptom onset, and management strategies, and multidisciplinary experience at a comprehensive pediatric epilepsy center is required to evaluate and treat affected children most effectively. The last several decades have seen significant improvement in the management of children with ETLE, and the field has enjoyed a shifting paradigm from one of watch and wait to one of earlier intervention that capitalizes on neural plasticity in the young child. Neural plasticity refers to the various mechanisms involved in the maturation of the developing cortex in children and the ability of the central nervous system (CNS) to respond to changes in its structure or function.5 Discoveries in the neuroscience community have fueled new data on neural plasticity and its impact on CNS development.6 Apoptosis, neurogenesis, synapse formation, and pruning all play a role in conferring a dynamic environment in the pediatric brain. Language dominance, for example, has been found to be set no earlier than 5 to 6 years of age.7,8 Neural plasticity plays a role in making possible a greater extent of surgical resection in eloquent cortex than would otherwise be permissible in adults. There is evidence that persistent seizure activity limits the degree of neural plasticity in the developing brain and may impact a child’s ability to achieve otherwise-anticipated neurocognitive outcomes.9 Although plasticity may allow the transfer of function from interhemispheric or intrahemispheric locations, neurocognitive development may be restricted to suboptimal levels.5 In a consideration of the clinical assessment and treatment options for pediatric patients with extratemporal etiologies, possibly with syndromic manifestations, it is necessary to keep plasticity in mind because it may substantially influence surgical outcomes.10 An estimated 65 million people are affected by epilepsy worldwide. The incidence in developed countries is nearly 50 per 100,000, and the prevalence is 700 per 100,000.11,12 As recognition of the morbidity associated with epilepsy improves, the disease is increasingly being diagnosed and evaluated by pediatric epilepsy teams. It is estimated that recurrent seizures affect approximately 1% of children,1 and these persistent seizures, if left untreated, are associated with long-term impairment in cognition, intelligence, and quality-of-life measures. It is estimated that 120,000 children in the United States will be evaluated each year for newly recognized seizures,13 and epilepsy will be diagnosed in as many as 45,000 of these cases. In addition, as many as 325,000 children ages 5 to 14 years have active epilepsy, and a majority of adults with epilepsy had a childhood onset.13 It is estimated that 100,000 to 200,000 patients in this country may be candidates for surgery because 10 to 20% of children with epilepsy meet the criteria for refractory epilepsy.14,15 Predictive factors for refractory epilepsy include early onset and an association with such syndromes as West syndrome, Lennox-Gastaut syndrome, and Ohtahara syndrome. More than 3,000 surgeries are performed each year in the United States for pediatric epilepsy, and with increasing recognition of the disease and the improving diagnostic tools available to surgeons and clinicians, this number is likely an underestimate.16 Before surgical management for ETLE is considered, otherwise treatable forms of epilepsy must be excluded.17 The first priority is to determine if adequate medication has been used to treat the epilepsy. Medication-refractory epilepsy is defined as epilepsy that persists or is unresponsive to two first-line antiepileptic drugs (AEDs).18,19 Despite optimal pharmacotherapy, the disease of 30% of the 65 million patients with epilepsy worldwide remains resistant to AEDs.20 The selection of AEDs is individually tailored to each child and takes into account the child’s developmental stage, the safety profile of the medication, and the presence of comorbidities. AEDs may affect cognitive and behavior functions, and their levels may be altered by other medications. Medications taken for comorbidities may lower the seizure threshold and impact AED selection.21 Children taking more than one AED to control their epilepsy are less likely to respond to additional medications, and once two or three medications have been attempted, benefit from pharmacotherapy is unlikely.5 Other predictors of drug resistance include mixed seizure types, neonatal age at onset, abnormal neurologic examination, early seizure breakthrough after treatment with medication, and underlying structural abnormalities.18 In addition, some children are unable to tolerate the side effects of medical treatment, and this, too, may confer a pharmacoresistant status. A ketogenic diet may be used to treat drug-resistant epilepsy in children. The ketogenic diet involves altering the ratio of fat intake to protein and carbohydrate intake. A ratio of 4 g of fat to every 1 g of carbohydrate and protein simulates the metabolic state of fasting and has been shown in a randomized controlled trial to significantly reduce more than 50% of seizures in selected pediatric patients.22 The ketogenic diet is the first line of treatment in epileptic disorders resulting from mutations in the genes for glucose transporter proteins and mutations that cause pyruvate dehydrogenase deficiency. The ketogenic diet is contraindicated in other metabolic disorders, such as those resulting from fatty acid oxidation or carnitine abnormalities.23 Dieticians and pediatricians can determine when such diet restrictions are warranted. Because some children who have epilepsy present with various syndromes, there may be additional risks and considerations that prevent surgical intervention. Cardiopulmonary and coagulation status and other comorbidities should not be excessive when surgical options are pursued. Finally, the results of a diagnostic work-up with various studies discussed in the following sections of this chapter should be concordant and implicate an epileptogenic region amenable to resection. The first step in identifying an epileptogenic zone for surgical resection is to use the information on seizure semiology to improve lateralization and localization.24,25 An assessment of semiology, or the observable manifestations of seizures, begins by combining historical information provided by the patient and family with video electroencephalographic (EEG) recordings. Descriptive terminology for seizure semiology has been introduced and is supported by the International League Against Epilepsy (ILAE) to improve the significance of localization and the recognition of semiologic features.26 Studies in pediatric patients have helped to identify semiologies that are common to anatomical regions or structures based on EEG findings and that are eradicated following surgical resections. Although misleading in certain cases, semiologies often provide additional clinical information for localizing ictal onset. Seizure semiologies may be difficult to detect in clonic, tonic–clonic, atonic, gelastic, and myoclonic seizures because they have a generalized onset and nonlocalizable features. These seizures may originate from subcortical regions, such as the thalamus, that generate synchronous activity with extensive ictal spread. A subset of generalized seizures may also have a focal onset that can be identified on EEG recordings, and definitive localization in these seizure types is difficult, so that additional diagnostic studies are warranted. There is also current debate about whether infantile spasms constitute a generalized or a focal seizure type.24 Seizures that involve sustained lateral deviation of the eye, head, or trunk from the midline are known as versive seizures and can indicate multilobar involvement. The following semiologies refer to an anatomical location; however, one-to-one correlation is not necessarily the rule because there may be degrees of overlap with adjacent cortical regions. The semiology of seizures may evolve as a child ages, and these changes must be considered. As the myelination of white matter pathways evolves in the first decade of life, intrinsic pathways develop to inhibit seizure propagation, causing the seizure semiology to more closely resemble adult patterns.27 Caution and confirmatory studies should be employed to identify concordant information for seizure localization. Seizures that lead to alterations in cognition, attention, memory, or other executive functions are often related to a temporal lobe origin. If the temporal lobe is involved unilaterally or bilaterally in seizure onset, there may be altered consciousness, verbal and nonverbal behavior, and postictal memory loss. In addition, temporal lobe seizures may present with various combinations of olfactory, gustatory, and pharyngeal auras. Auras may also originate from multilobar involvement. Other aura types, such as visual hallucinations, are associated with involvement of the parietal and/or occipital lobes. Finally, oral and upper extremity automatisms may also originate from the temporal lobe, whereas other automatisms, such as bicycling, may be related to frontal lobe pathology. Frontal lobe seizures are the most common ETLE and account for approximately 20 to 30% of childhood epilepsies.28 Frontal lobe seizures also comprise a significant portion (15 to 30%) of medication-resistant epilepsies. Seizures in the frontal lobe have far-reaching effects and may manifest with a host of possible deficits in children. Children who have frontal lobe epilepsy present with impaired motor and executive function and with socialization, behavior, speech and language, and cognition problems. Behavioral dysfunction manifests as disinhibition, aggression, or attention disorders. Additionally, when children who have frontal lobe epilepsy are compared with patients who have temporal lobe–based or absence seizures, the children with frontal lobe ictal zones have more difficulty with perceptual organization, self-regulation of behavior, impulse control, visual and auditory attention, and visual working memory.29 Frontal lobe seizures present with variable semiologies that include hypermotor activity with repetitive movements in the upper extremities and can be associated with loss of consciousness. Hypermotor activity in children is also a prominent component of seizures originating in the temporal lobe and may decrease with age. In contrast, diminished motor activity, or hypomotor seizures, tends to arise from temporal or parietal locations.24 In children younger than 7 years, frontal lobe epilepsy presents with subtle behavioral changes that differ from those in adolescents or adults. Frontal lobe seizures may also present with a diffuse warm feeling throughout the body or as an altered sensation in the body. Postictal wiping of the nose can occur in patients with frontal lobe seizures; however, this is more common in those with seizures of temporal lobe origin.30 Benign rolandic epilepsy is one of the most common childhood epilepsies, with an estimated incidence of 5 to 15% of pediatric epilepsies.31 It is characterized by transient simple partial seizures with hemiparesis, facial palsies, difficulty swallowing, hypersalivation, and oromotor apraxia.32 These seizures have a typical presentation pattern in children 3 to 10 years of age and may spontaneously remit by adolescence. The seizures frequently occur during sleep or shortly after awakening, and neurocognitive studies are often normal in these children.31 Multidisciplinary epilepsy teams, however, are becoming increasingly aware of a certain population of children in whom rolandic seizures were previously diagnosed who present at earlier ages (younger than 6 years) and have progressive cognitive impairments. These children are diagnosed with malignant rolandic epilepsy33 that is refractory to antiepileptic medications and whose semiology may change. The term malignant is used to identify the increased frequency and clustering of seizures, resistance to medical therapy, and longer duration of the disorder. The seizures may also involve somatosensory symptoms and can generalize to tonic–clonic seizures.32,34 Parietal lobe seizures account for only 5% of partial seizures despite their large cortical volume.35 The semiology of the parietal lobe is difficult to detect because epileptic activity may rapidly spread from ictal foci to adjacent lobes, triggering events that are seen as originating from the temporal or occipital region.36 The parietal lobe subserves several eloquent cortical functions, including integration of visual information, language, praxis, and attention. Parietal lobe damage results in apraxia, agnosia, agraphia, acalculia, left–right disorientation, hemineglect, and altered body image.37 Parietal lobe seizures involve visual or somatosensory auras that can be localized to a particular region of the patient’s body.38 In addition, parietal lobe seizures present with ictal pain, complex visual hallucinations, and autoscopy (out-of-body experience).36 Seizures originating from the parietal region can quickly spread to the temporal lobe and present with semiologies similar to those of temporal lobe epilepsy. Occipital lobe epilepsies account for an estimated 8 to 28% of partial epilepsies in children,38–40 and some of the earliest reports of seizure semiology in occipital lobe epilepsy were described in 1885.41 A large number of patients will have Panayiotopoulos syndrome, the second most common childhood epilepsy after benign childhood epilepsy, and do not require surgical intervention because there is a significant rate of spontaneous seizure remission in these patients.42 It is estimated that only 2% of epilepsy procedures are done in children with occipital lobe seizures.39 The ILAE has divided occipital lobe epilepsies into two types depending upon early or late onset. Early-onset seizures occur at a median age of 5 years and present with nonvisual symptoms, such as vomiting, eye deviation, and tonic head movements. Late-onset seizures occur at a mean age of 9 years and most commonly involve visual hallucinations, followed by illusions, palinopsia (persistence of an image or its recurrence), and visual auras, as well as ictal blindness and amaurosis with loss of portions of the visual fields.40,43 If the seizures spread, temporal lobe automatisms or focal motor seizures may develop if the supplementary motor cortex is affected.39 Children with occipital lobe seizures are also found to have lower verbal and performance IQs and can be at increased risk for neurobehavioral disorders.42 The diagnostic work-up required for children with ETLE has become more advanced throughout its evolution and now includes a host of technologies routinely used by epilepsy centers to identify the etiology of seizures and ictal onset.44 The utilization of each test depends largely upon the suspected etiology, and tests may proceed to more advanced technology if the information is discordant. Adding a further layer of complexity to the analysis of children with ETLE is the changing nature of seizure types, as in infants who present with generalized or partial seizures that can evolve to complex partial seizures, absence, generalized tonic–clonic, or myoclonic seizures. EEG serves as the foundation of inquiry into epilepsy in children. Video EEG units have the added power of coupling longitudinal electrophysiologic data with clinical manifestations to determine the effect of a seizure on a child’s function. Interictal epileptiform discharges (IEDs) often originate from an area of the cortex that is somehow associated or responsible for ictal discharges. In temporal lobe epilepsy, these discharges can be associated with mesial temporal sclerosis or temporal tumors. In ETLE, the use of EEG to identify focal IEDs, such as those seen with focal cortical dysplasia (FCD), is associated with improved surgical outcomes for seizure-free activity. Ictal EEG recordings are helpful for localization in cases with a high degree of recording concordance. Extra attention must be paid in cases of ETLE in which parietal and occipital lobe seizures can cause a false localization. ETLE in the frontal lobe can frequently present with focal rhythmic fast activity on EEG. Scalp EEG source localization is an additional technique used to identify the epileptic center of activity and may be of use in preoperative studies of ETLE.45 Although EEG remains the cornerstone for the evaluation of ETLE, it has limitations when pediatric patients present with the synchronous onset of epileptiform activity over a diffuse epileptogenic area, which represents multilobar pathology. Additionally, there can be poor localization with EEG, an absence of IEDs, or no appreciable EEG changes with clinical seizure onset. These limitations reduce the likelihood of identifying the ictal onset in ETLE and warrant the performance of additional diagnostic studies. High-frequency oscillations (100 to 500 Hz) are associated with epileptic regions in the hippocampus and neocortex46 and may be used as biomarkers for epileptogenic regions.47 Prospective studies to correlate high-frequency oscillation rates with zones of seizure onset are ongoing and will determine the predictive value of this technology.48,49 Neuroimaging modalities and their uses in diagnosing the etiologies of pediatric ETLE have seen significant changes over the past 20 years and may be the most important sources of improved presurgical planning in children with ETLE. With improved resolution, magnetic resonance (MR) imaging has helped to detect previously unidentified brain lesions. Field strengths in excess of 1.5 tesla (T) and phased-array coils are increasingly being used for volumetric studies and localization in a clinical setting.50 The use of 3-T imaging increased the identification of lesions in 65% of patients previously reported to have negative images.51,52 Although lesions are more clearly viewed, their contribution to the onset or persistence of epileptiform activity must be correlated with clinical and electrophysiologic data, such as EEG recordings. Seizure-free outcomes are improved when EEG and neuroimaging modalities are concordant.53,54 If, however, the patient has multiple lesions or other associated malformations of cortical development (MCDs), additional evaluation with diagnostic studies is warranted. Despite the increasing reliance on MR imaging to localize lesions, seizure freedom has been successfully achieved55 in patients in whom no appreciable lesion could be identified on MR imaging.56,57 The use of intracranial EEG (iEEG),9 positron emission tomography (PET), and ictal single photon emission computed tomography (SPECT) improved surgical outcomes in 75% of patients, with a 37% seizure-free rate in cases in which MR imaging was unsuccessful in localizing a lesion. Surgery remains a treatment option even when the results of MR imaging are equivocal. Questions arise about which pediatric patients should be screened with MR imaging. When a child presents with a first-time seizure, computed tomography (CT) is usually performed in the emergency department, with one-third of these patients later determined to have epilepsy. Previous studies have shown that 7 to 24% of CT procedures identify abnormalities warranting management.58 MR imaging is the next imaging modality for evaluating children; however, 50% of imaging studies in patients with idiopathic generalized epilepsy are normal. The threshold to obtain MR imaging in infants is lower because they are at an increased risk for developmentally related malformations that may manifest without otherwise localizable features.54 Children who should also undergo early MR imaging are those with focal seizures, abnormal or focal neurologic examinations, developmental delay or regression, generalized epilepsy, signs of increased intracranial pressure, or syndromic manifestations or family histories of epilepsy.58 Pediatric neuroradiologists familiar with developmental changes in the appearance of the cortex are required for the optimal screening of MR imaging findings.52 Many protocols have been developed by various centers to optimally image pediatric epilepsy-related pathologies. Most surgical centers require thin-slice volumetric T1-weighted images with and without contrast, T2-weighted images, and FLAIR (fluid-attenuated inversion recovery) images in an axial, coronal, and sometimes sagittal reconstruction. Volumetric MR images have also been useful to identify hippocampal volumes to determine if a component of mesial temporal lobe sclerosis is present. MR imaging is paired with MR spectroscopy to identify markers of neuronal loss and the presence of certain tumors or other abnormalities. MR imaging has helped not only to identify congenital MCDs, tumors, and other abnormalities, but also to confirm in an intraoperative setting the complete resection of lesions, thereby reducing the need for additional surgery.59 Still, the lesions of 20 to 30% of patients are not localized with MR imaging.60 As the number of cryptogenic cases of ETLE increases at pediatric epilepsy centers, newer MR technologies are pushing the boundaries to identify underlying lesions. These techniques are being applied primarily to FCDs that result from abnormal cortical development and migration defects. Structural MR imaging is employed to look for subtle abnormalities in gyral and sulcal morphometry. Voxel-based intensity and morphometric studies are used along with quantitative image analysis61 to identify lesions through automated pattern recognition.57 Diffusion tensor imaging (DTI) and tractography rely on the anisotropic diffusion of free water to identify white matter tract organization. The use of DTI for preoperative planning62 is rapidly expanding, and DTI has aided in the identification of optic tract,63 motor strip, and other eloquent cortical involvement in and around epileptogenic foci. DTI can also reveal changes that occur in patients with certain pathologies64 or with language and memory impairments, and the results can be correlated with neurocognitive outcomes.65 DTI is used to obtain high-resolution functional and structural data that can guide the stereotactic placement of subdural EEG electrodes in ETLE cases.66 DTI improved surgical decision making in 66% of patients, and its use is correlated with improved surgical outcomes for ETLE.67 Following surgery,68 DTI may also be employed to assess the plasticity of the underlying cortex and remodeling in fiber bundles.69 Functional MR imaging (fMRI) is an additional use of MR imaging that maps cerebral activity by detecting changes in blood flow while a patient performs associated tasks. fMRI works by detecting the level of deoxygenated hemoglobin, which decreases when a cortical area is activated, thereby increasing blood flow to that cortex.70 This technique, called blood oxygenation level–dependent (BOLD) contrast fMRI, has been useful in mapping language function.71 fMRI can be successfully performed in children as young as 5 years of age in whom the lateralization of motor and other functional capacities has already been established.27,58 fMRI techniques are used to lateralize language function in the temporal lobe to aid in preoperative evaluation.72 fMRI can also be used in preoperative studies to assess motor tasks.73,74 Recent advances in fMRI use spontaneous BOLD fluctuations to map functional connectivity in the brain. A normal brain that is not performing a task exhibits slow (< 0.1 Hz) fluctuations in the BOLD signals that correlate with neuronal activity75 in a distinct functional network. Functional connectivity MR imaging (fcMRI) can be performed in children at rest to identify sensorimotor and language cortical regions. There are several advantages to using fcMRI in children because they are not required to perform an age-dependent or cognitive-dependent verbal or motor task. Thus, children may be investigated at earlier ages. In addition, a single study will allow the deciphering of various functional connectivity networks with improved signal-to-noise ratios.76 The future use of fcMRI in preoperative evaluations may improve our understanding of anatomical connectivity and function in pediatric ETLE.77 PET is increasingly being used to determine areas of ictal onset by identifying hypometabolic regions corresponding to abnormal cortex.78 PET uses fluorodeoxyglucose (FDG) to approximate metabolic activity in the cortex. Hypometabolic FDG-PET has been used to identify and localize regions of suspected epileptogenesis that require additional investigation. In addition, α-methyl tryptophan (AMT)-PET is used to differentiate between epileptogenic and nonepileptogenic lesions in patients with tuberous sclerosis and MCDs. Finally, flumazenil (FMZ)-PET79 can identify epileptic cortex beyond an abnormality on MR imaging by detecting areas with decreased binding to γ-aminobutyric acid A receptors.1 PET has the advantage of using different receptor binding sites in the brain, which facilitates ongoing investigations into the neurobiology of epileptogenesis.78 SPECT approximates perfusion to a suspected area of cortical involvement in epileptiform activity following the injection of a radioactive tracer. Focal increases in perfusion reflect epileptic activity. Ictal SPECT measurements approximate metabolic demands and may localize hypermetabolic lesions like FCD when conventional MR imaging is equivocal. Studies can be compared in which subtracted ictal–interictal SPECT data can increase the sensitivity for detecting differences between perfusion rates in the ictal and interictal states. The resulting information can be co-registered with MR imaging (subtraction of interictal from ictal SPECT co-registered to three-dimensional MR imaging, or SISCOM) to identify epileptic foci.80 In cases of tuberous sclerosis81 and other multifocal abnormalities, SPECT is especially useful for defining the epileptogenic zone. Co-registration with MR imaging can also be employed to guide and implant subdural electrodes. Ictal SPECT has limitations, however, because it is difficult to synchronize the timing of the injection radioactive tracer with ictal onset, and close video EEG and clinical monitoring by a trained multidisciplinary epilepsy team are required. MEG is a more recent technology that is increasingly used to identify magnetic fields and dipole clusters produced by cortex with interictal epileptiform activity. In turn, MEG can detect electrical activity that is parallel to the cortex and tangential to the convexity.82 The magnetic fields are not dissipated by the intervening structures of the skull and scalp, which aids in localization. MEG results have been verified by correlation with iEEG recordings and have improved localization in equivocal cases in which video EEG and MR imaging appeared to be discordant. The accuracy of MEG for predicting surgical outcome was compared with that of MR imaging and various EEG techniques and was found to be second only to that of intracranial subdural EEG monitoring.83,84 In a study of ETLE, concordance between MEG and iEEG data in localizing epileptiform discharges corresponding to the ictal zone was found in 91% of children.85 The future applications of MEG may reduce the number of patients requiring invasive studies, leading to a more cost-effective and safe use of diagnostic studies in children.86 Failure to remove regions with active preoperative MEG dipoles predicts postoperative seizure recurrence.87,88 MEG is being increasingly used to reduce the number of studies required to evaluate infants undergoing hemispherectomy.89 Some epilepsy centers are turning to MEG as a primary modality to identify the ictal area during preoperative planning and resection.90 MEG has the ability to map sensorimotor, language, and other functional capacities, and its accuracy is comparable to the perceived gold standard of iEEG monitoring.90 In magnetic source imaging (MSI), MEG and MR imaging data are combined to optimize the results of each modality for structural and functional data. Three-dimensional images can be generated to delineate the morphological and functional changes wrought by epileptogenic tissue.91 Epileptic foci can be accurately pinpointed with this technique and compared with eloquent cortical regions or detectable structural abnormalities.92 This information can guide surgical resections or lead to palliative procedures, such as multiple subpial transections. MSI correlates with surgical findings in more than 89% of cases93 and has a reported accuracy of 100% in ETLE cases.91 MSI improved localization data in 35% of patients and altered treatment decisions in 10% of patients. In cases of tuberous sclerosis, MSI has a reported 100% sensitivity, 94% specificity, and 95% accuracy in identifying epileptogenic lesions in children.94 MSI data can also be used to predict surgical outcomes.31,95 The intracarotid injection of sodium amobarbital (Wada test) is used to study the function of a cerebral hemisphere. Following injection, the ipsilateral hemisphere is temporarily inactivated, and language and memory function in the contralateral hemisphere can be tested to localize these functions. Wada testing is limited because it is invasive, and it may be supplanted by fMRI or MEG in the future. There is growing recognition that seizure freedom is not the only measure of a successful outcome postoperatively. A number of neurocognitive tests have been developed to monitor preoperative deficits and postoperative outcomes, with the goal of improving the pediatric patient’s quality of life following intervention. Each patient undergoing surgical evaluation requires a complete neurocognitive evaluation to identify cognitive function, language skills, intelligence, attention, memory, executive function, personality, behavior, and motor and sensory limitations. Children with epilepsy have a higher rate of comorbidities when compared with control groups. Childhood comorbidities in epilepsy include attention-deficit/hyperactivity disorder (ADHD), autism, depression, anxiety, developmental delay, and cognitive and behavioral abnormalities. Symptoms of ADHD were identified in 38% of children with epilepsy, and depression and anxiety were seen in 26% and 16% of epileptic children, respectively.96 Epilepsy is found in 28% of autistic children.13 Finally, the incidence of mental retardation and cerebral palsy is upward of 38% in children with epilepsy.97 Despite extensive evaluation and a combination of various noninvasive modalities to localize the epileptogenic region, a considerable fraction of children with medically refractory ETLE will require iEEG monitoring with invasive techniques. These patients often have normal or nonlocalizing imaging, widespread EEG abnormalities, or multiple lesions. To pursue subdural monitoring with grids, strip, and depth electrodes, input from an experienced epilepsy team is warranted. Seizure freedom is the ultimate goal in a neurosurgical intervention for pediatric epilepsy. In some cases this may be an unachievable goal, however, and palliative procedures are offered to reduce the seizure burden. Managing the expectations of families adds a further layer of complexity to the treatment of ETLE.98 As the scientific and clinical communities have increasingly recognized the difficulties associated with ETLE treatment, there is a preferred exclusion of patients based on extratemporal locations.99 To obviate such an approach, patient selection and knowledge of the various syndromes and seizure types become essential components of surgical planning and decision making.100 Parental angst may be offset by the collective experience offered by pediatric epilepsy centers for difficult cases. Multidisciplinary meetings with epileptologists, neurosurgeons, radiologists, EEG technicians, neuropsychologists, pediatricians, therapists, and other associated healthcare providers facilitate the summation of the information collected for each child who is a potential surgical candidate. During these meetings, the diagnostic information obtained with video EEG, semiology, and neuroimaging, together with potential surgical planning, is used to frame possible solutions to reduce or eliminate seizure burden. As a result of collective experience and discussions, aided by improved diagnostic techniques, increasing numbers of children are being considered for surgical intervention. Cost analysis in developing countries reveals that lesions can be cost-effectively treated with limited resources. Ideal patients are those with lesions identified by MR imaging that have been co-localized with interictal and ictal EEG.99 With a multidisciplinary approach and the success of surgery in ETLE, there is a growing trend to identify children as early as possible for surgical intervention and to select patients who are at high risk for failing medical therapies. The early identification of surgical candidates is one goal of surgical centers, where children can be evaluated expeditiously instead of waiting for prolonged unsuccessful medical treatments. There is a balance that must be struck in the early identification of children with surgically amenable pathologies. Adequate medical therapy should be advocated while the selection of surgical candidates is improved. Children with infantile spasms, multiple seizure types, or recurrent seizures within 1 year of treatment are likely to progress to intractable epilepsy that may also require surgical intervention and are less likely to respond to medical therapies.27 Patients who have syndromes associated with ETLE may also benefit, with surgery considered for those with Sturge-Weber syndrome, tuberous sclerosis, FCD, hemimegalencephaly, Rasmussen syndrome, or low-grade tumors. With a greater understanding of seizure types and the neurodevelopmental burden of seizures, it is clear that more children can now be helped.8,101 There are also patient variables that make seizure-free outcomes less likely in surgical candidates. Diffuse lesions on MR imaging, seizures with a neonatal onset, a longer duration of epilepsy, psychiatric comorbidities, and IQs below 70 have all been associated with poorer postoperative outcomes. Patients undergoing multilobar resections are also less likely to achieve seizure-free status. In addition, the histopathologic diagnosis of a tumor is associated with an improved surgical outcome in comparison with FCD.102 The seizure-free outcomes of FCD type II may be better than those of type I as a result of improved visualization with MR imaging to aid complete surgical resection. Epilepsy teams are also charged with evaluating the pediatric patients in follow-up, looking not only for degree of seizure remission or freedom but also for neurocognitive outcomes and, ultimately, the ability to function independently in society. The surgical resection of epileptic foci in pediatric patients began with Victor Horsley in 1886.8 Initial results from these efforts suggested a reduction in seizure frequency and tolerable clinical outcomes. With the later availability of EEG, Wilder Penfield pursued epilepsy surgery at the Neurological Institute in New York and later founded the Montreal Neurological Institute while pioneering the use of electrocorticography (ECoG) to guide surgical resections of epileptogenic tissue.103 During his studies, Penfield focused primarily on adult pathology and temporal lobe epilepsy, but he also performed a significant number of surgeries for ETLE.104–109 This and other groundbreaking work slowly lead to the expansion of surgical techniques in children.110,111 Continued progress in clinical studies in the 1980s and 1990s led to the increasing appreciation that younger patients with ETLE were surgical candidates. While hemispherectomy and other disconnection surgeries were gradually adopted into the realm of pediatric epilepsy, a host of new surgical techniques, paired with advancements in diagnostic techniques, paved the way for extratemporal surgery in a pediatric population. The most straightforward application of surgery for the treatment of ETLE in children is single-stage surgery for lesionectomy or lobectomy. When the aforementioned diagnostic tools (MR imaging, EEG and clinical data) are concordant in their localization of a lesion responsible for seizures, surgical resection is pursued. Children with a resectable offending lesion have the best seizure-free outcomes, with results related to a gross total resection. Advanced diagnostic tools like PET and SPECT may be avoided in children in whom concordant results identify a single lesion. During surgery, ECoG is often used to identify interictal data and functional cortical regions. ECoG remains the gold standard for the evaluation of an ictal onset and can be performed during surgical resection, after the implantation of subdural grids, or following surgical resection to evaluate areas for ongoing epileptiform activity. General anesthesia without benzodiazepines is preferable.112 A larger craniotomy will allow mapping of the cortical surface. Depth electrodes may be placed with frame-based or frameless stereotactic systems that can also be used to guide surgical resection. ECoG requires that grounding and reference electrodes be placed subgaleally or on the scalp surface. Cortical mapping is possible during surgery with somatosensory evoked potentials (SSEPs) and bipolar cortical stimulation.113 Low stimulus settings can be used to avoid eliciting seizures and may be tailored according to the age of the patient. Settings for cortical stimulation may include 3- to 5-mA, 50-Hz biphasic square wave pulses with a duration of 0.3 millisecond.114 The current can be increased in small intervals until an ictal event or afterdischarge is seen or if a current of 15 to 16 mA is reached. Likewise, the pulse duration may be increased in a stepwise manner until a duration of 1 millisecond is achieved. The central sulcus may be identified by phase reversal in SSEPs, and motor evoked potentials may be used to further identify regions of the motor cortex. The response to motor cortex stimulation is related to the age of the patient, with infants showing no response given the underdeveloped myelination of the major motor pathways. Seizures that are elicited on ECoG can be aborted with the application of cold saline to the cortical surface.115,116 After ECoG and cortical mapping, resection of the lesion is pursued while the boundaries of eloquent cortex are respected. Multiple subpial transections, discussed later in this chapter, may be used if the ictal region involves eloquent cortex. Efforts should be made to avoid the retraction of normal brain to avoid vascular compromise, infarct, and postoperative edema. If a lesion is present, ultrasound or frameless MR imaging neuronavigation may aid in identifying the limits of surgical resection, and a lesionectomy can be performed. If only epileptogenic cortex is present, a corticectomy is performed, in which the gray matter is removed with a subpial dissection technique down to the white matter. Care is taken to follow the gyral folds to the depths of the sulcus to avoid leaving epileptic tissue behind. ECoG after resection allows confirmation of complete resection of the ictal focus. Postoperatively, anticonvulsants are continued according to the patient’s previous home regimen and may be tapered based on close observation by pediatric neurologists. The current technology of diagnostic imaging and electrophysiology can be stretched to its limits as the complexity of cases addressed by pediatric epilepsy teams (younger ages of patients, increased comorbidities, syndromic findings) increases. Frequently, discordant data cloud localization of the area of ictal onset. One technique that improves a neurosurgeon’s ability to diagnose and treat ETLE is the use of subdural electrodes for neurophysiologic recordings and ECoG.117 The implantation of electrodes for iEEG monitoring has gained popularity in the last decade, largely to address the number of patients who have discordant diagnostic studies or who have lesions adjacent to eloquent cortex.32,118 The benefit of iEEG is that children who were previously not surgical candidates can now undergo invasive studies so that a surgical approach can be tailored to their particular underlying pathology. iEEG with electrodes allows longitudinal monitoring, which can better identify epileptogenic cortex and further map eloquent areas. Ictal and interictal activity can be studied, and cortical stimulation can be employed to identify motor, speech, and language areas in children who are of an age to have these localizable functions identified. In addition, stereotactically placed depth electrodes are used to probe subcortical regions where activity is not otherwise recorded by superficial grid arrays.119,120 Neuronavigation with frame or frameless stereotactic systems is also routinely employed to take advantage of MR imaging that has been fused with MEG, PET, and/or SPECT data. The combination of neuronavigation with these diagnostic modalities improves surgical planning and identifies nearby eloquent cortex that limits resection. The first stage of surgery involves using the aforementioned diagnostic studies to identify areas of interest either in one or both hemispheres that are candidates for iEEG monitoring. Preoperatively, epileptologists and neurosurgeons can design an optimal array of strip, grid, and depth electrodes to obtain the necessary electrophysiologic information and to maximize coverage of the cortical surface (▶ Fig. 73.1a). Detailed thin-cut MR imaging with venography or arteriography can aid in surgical planning, especially if stereotactic localization is used. Subdural grids are especially useful for assessing the interhemispheric cortex, orbital frontal cortex, mesial temporal lobe, and cingulate gyrus. The implanted arrays are silicon-covered platinum–indium electrodes that are MR compatible and allow postoperative imaging to confirm anatomical localization (▶ Fig. 73.1b,c). After placement of the subdural electrodes, epilepsy teams will note the location and orientation of the arrays, often with intraoperative photographs for later matching to imaging and iEEG data (▶ Fig. 73.1d). To augment the information offered by surface array coverage, depth electrodes can safely be employed in cases of ETLE to target cortex that may lie tangentially to conventional strip or grid electrodes (▶ Fig. 73.2a). The electrophysiology of sulci and regions like the interhemispheric cortex and frontal and temporal opercular cortex may also probed (▶ Fig. 73.2b) with depth electrodes.121 Fig. 73.1 Ictal localization for a parieto-occipital resection. (a) Pictorial representation for planning the placement of the grid array with respect to the cortical surface. The 8 × 8 grid can be seen, with adjacent six-lead strip electrodes overlying various portions of the cortex. The central sulcus is marked under the overlying grid. SSEP, somatosentory evoked potential. (b) Postoperative lateral plain radiograph revealing placement of the array of grid and strip electrodes for ictal localization. The underlying craniotomy can be seen with lucency in the calvaria. (c) Postoperative anteroposterior plain radiograph revealing the same placement of the grid array and strip electrodes. (d) Electroencephalogram from placement of the grid and electrodes localizing epileptic activity with spike–wave complexes to the occipital lobe.
73.1 Neural Plasticity
73.2 Epidemiology: Incidence and Prevalence
73.3 Medical Treatment and Definitions of Refractory
73.4 Common Semiologies by Lobe
73.4.1 Temporal Lobe Seizures
73.4.2 Frontal Lobe Seizures
73.4.3 Parietal Lobe Seizures
73.4.4 Occipital Lobe Seizures
73.5 Diagnostic Work-up
73.5.1 Noninvasive Electroencephalography and Video Electroencephalography
73.5.2 Neuroimaging
73.5.3 Functional Magnetic Resonance Imaging
73.5.4 Functional Connectivity Magnetic Resonance Imaging
73.5.5 Positron Emission Tomography
73.5.6 Single Photon Emission Computed Tomography
73.5.7 Magnetoencepholography and Magnetic Source Imaging
73.5.8 Wada Test
73.5.9 Neurocognitive Testing
73.6 Surgical Decision Making: Epilepsy Center Structure
73.7 Surgery
73.7.1 Single-Stage Procedures
73.7.2 Two-Stage Procedures
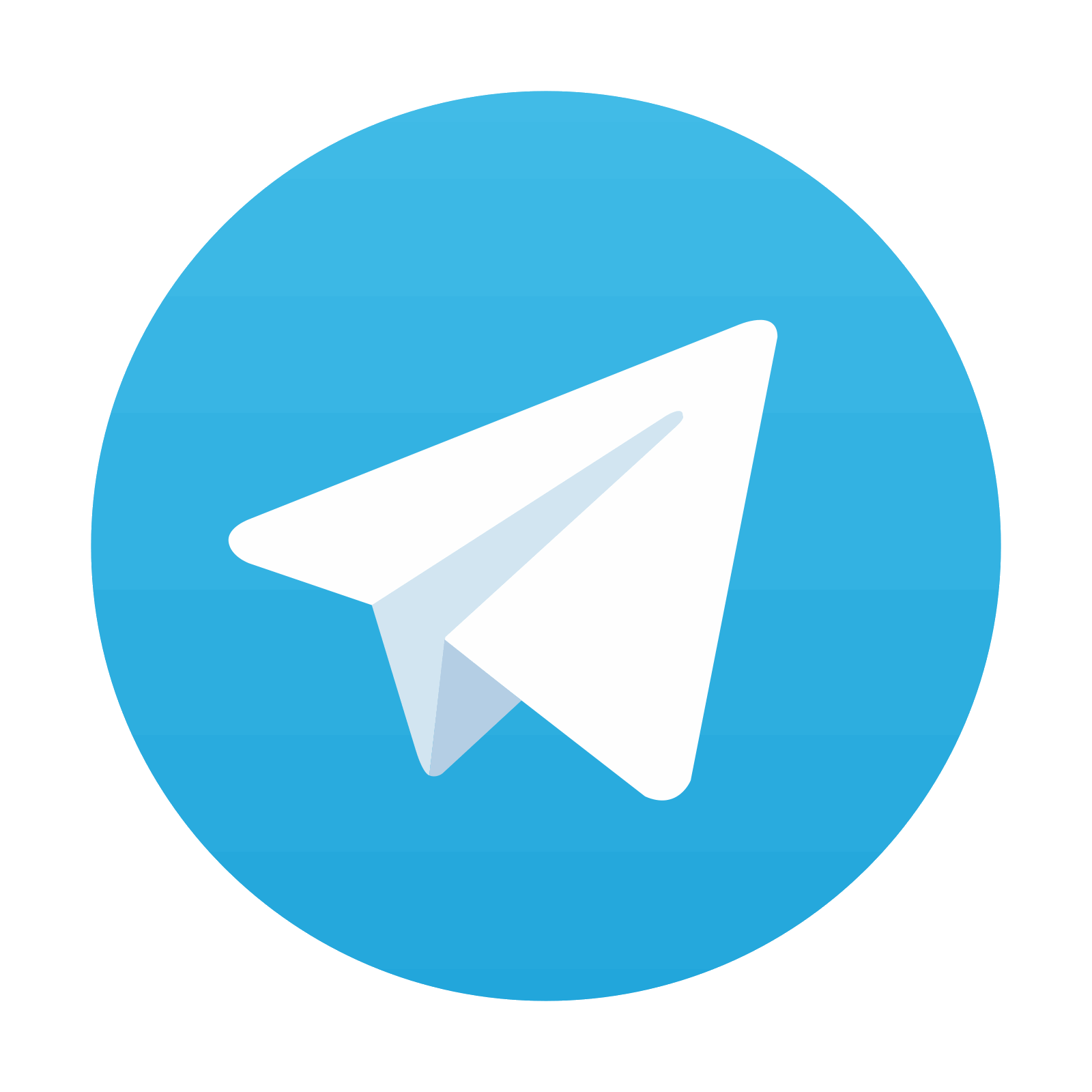