Formation and Elimination of Synapses
Recognition of Synaptic Targets Is Specific
Different Synaptic Inputs Are Directed to Discrete Domains of the Postsynaptic Cell
Principles of Synaptic Differentiation Are Revealed at the Neuromuscular Junction
Differentiation of Motor Nerve Terminals Is Organized by Muscle Fibers
Differentiation of the Postsynaptic Muscle Membrane Is Organized by the Motor Nerve
The Nerve Regulates Transcription of Acetylcholine Receptor Genes
Central Synapses Develop in Ways Similar to Neuromuscular Junctions
Neurotransmitter Receptors Become Localized at Central Synapses
Synaptic Organizing Molecules Pattern Central Nerve Terminals
Some Synapses Are Eliminated After Birth
SO FAR WE HAVE EXAMINED THREE STAGES in the development of the mammalian nervous system: the formation and patterning of the neural tube, the birth and differentiation of neurons and glial cells, and the growth and guidance of axons. One additional step must occur before the brain becomes functional: the formation of synapses. Only when synapses are formed and functional can the brain go about the business of processing information.
Three key processes drive synapse formation. First, axons make choices among many potential postsynaptic partners. By forming synaptic connections only on particular target cells, neurons assemble functional circuits that can process information. Usually synapses must be formed at specific sites on the postsynaptic cell; some axons form synapses on dendrites, others on cell bodies, and yet others on axons or nerve terminals. Cellular and subcellular specificity are evident throughout the brain, but we will illustrate the general features of synapse formation with a few well-studied classes of neurons.
Second, after cell-cell contacts have formed, the portion of the axon that contacts the target cell differentiates into a presynaptic nerve terminal, and the domain of the target cell contacted by the axon differentiates into a specialized postsynaptic apparatus. Precise coordination of pre- and postsynaptic differentiation depends on interactions between the axon and its target cell. Much of what we know about these interactions comes from studies of the neuromuscular junction, the synapse between motor neurons and skeletal muscle fibers. The simplicity of this synapse made it a favorable system to probe the structural and electrophysiological principles of chemical synapses, and this simplicity has also helped in the analysis of developing synapses. In this chapter we use the neuromuscular synapse to illustrate key features of synaptic development, and we also apply insights from this peripheral synapse to examine the synapses that form in the central nervous system.
Finally, once formed, synapses continue to mature, often undergoing major rearrangements. One striking aspect of later development is the wholesale elimination of a large fraction of synapses, a process that is usually accompanied by the growth and strengthening of surviving synapses. Like neuronal cell death (see Chapter 53), synapse elimination is a puzzling and seemingly wasteful step in neural development. It is increasingly clear, however, that it plays a key role in refining initial patterns of connectivity. We will discuss the main features of synapse elimination at the neuromuscular junction, where it has been studied intensively, as well as at synapses between neurons, where it also is prominent.
Throughout this chapter we emphasize the interplay of molecular programs and neural activity in shaping synaptic patterns. Synapse formation stands at an interesting crossroads in the sequence of events that assemble the nervous system. The initial steps in this process appear to be “hardwired” by molecular programs. However, as soon as synapses form, the nervous system begins to function, and the activity of neural circuits plays a critical role in subsequent development. Indeed, the information-processing capacity of the nervous system is refined through its use, most dramatically in early postnatal life but also into adulthood. In this sense the nervous system continues to develop throughout life. This discussion will be a useful prelude to Chapter 56, where we discuss how genes and the environment—nature and nurture—interact to customize nervous systems early in postnatal life.
Recognition of Synaptic Targets Is Specific
Once axons reach their designated target areas they must choose appropriate synaptic partners from the many potential targets within easy reach. Although synapse formation is thought to be a selective process at both cellular and subcellular levels, few of the molecules that confer synaptic specificity have been identified.
Recognition Molecules Promote Selective Synapse Formation
The specificity of synaptic connections is particularly evident when intertwined axons select subsets of target cells. In these cases axon guidance and selective synapse formation can be distinguished. The first report of such specificity came more than 100 years ago when J. N. Langley, studying the autonomic nervous system, proposed the first version of a chemospecifi-city hypothesis (see Chapter 54).
Langley observed that autonomic preganglionic neurons are generated at distinct rostrocaudal levels of the spinal cord. Their axons enter sympathetic ganglia together but form synapses with different postsynaptic neurons that innervate distinct targets. Using behavioral assays as a guide, Langley inferred that the axons of preganglionic neurons located in the rostral spinal cord form synapses on ganglion neurons that project their axons to relatively rostral targets such as the eye, whereas neurons that derive from more caudal regions of the spinal cord synapse on ganglion neurons that project to caudal targets such as the ear (Figure 55-1A). He then showed that similar patterns were reestablished after the preganglionic axons were severed and allowed to regenerate, leading him to postulate that some sort of molecular recognition was responsible (Figure 55-1B, C).
Figure 55-1 Preganglionic motor neurons regenerate selective connections with their sympathetic neuronal targets.
A. Preganglionic motor neurons arise from different levels of the thoracic spinal cord. Axons that arise from rostrally located thoracic neurons innervate superior cervical ganglion neurons that project to rostral targets, including the eye muscles. Axons that arise from neurons at caudal levels of the thoracic spinal cord innervate ganglion neurons that project to more caudal targets, such as the blood vessels of the ear. These two classes of ganglion neurons are intermingled in the ganglion, which suggested to J. N. Langley that preganglionic axons from different thoracic levels selectively form synapses with neurons that terminate in specific peripheral targets.
B. After nerve damage in adults, similar segment-specific patterns of connectivity are established through reinnervation, supporting the notion that synapse formation is selective. (Reproduced, with permission, from Nja and Purves 1977.)
Electrophysiological studies later confirmed Langley’s intuition about the specificity of synaptic connections in these ganglia. Moreover, this selectivity is apparent from early stages of innervation, even though specific types of postsynaptic neurons are interspersed within the ganglion. The reestablishment of selectivity in adults after nerve damage shows that specificity does not emerge through peculiarities of embryonic timing or neuronal positioning.
To illustrate the idea of target specificity in more detail we will consider the axons of developing retinal ganglion cells. These neurons differ in their response properties—some ganglion neurons respond to increases in light level (ON cells), others to decreases (OFF cells), others to moving objects, and still others to light of a particular color. The axons of all ganglion cells run through the optic nerve, forming parallel axonal pathways from the retina to the brain.
The response properties of each class of ganglion neurons depend on the synaptic inputs they receive from amacrine and bipolar interneurons, which in turn receive synapses from light-sensitive photoreceptors. All of the synapses from bipolar and amacrine cells onto ganglion cell dendrites occur in a narrow zone of the retina called the inner plexiform layer (see Chapter 26). Axons and dendrites therefore have the daunting task of recognizing their correct partners within a large crowd of inappropriate bystanders.
In the inner plexiform layer the processes of different amacrine and bipolar cell types, as well as the dendrites of functionally distinct ganglion cell types, branch and synapse in different sublayers. The dendrites of ON and OFF cells are restricted to inner and outer portions of the plexiform layer, respectively, and therefore receive synapses from different interneurons (Figure 55-2). There are a dozen sublayers within the inner plexiform layer, each with its own cohort of synapses. Similar lamina-specific connections are found in many other regions of the brain and spinal cord. For example, in the cerebral cortex distinct populations of axons confine their dendritic arbors and synapses to just one or two of the six main layers.
Figure 55-2 Retinal ganglion neurons form layer-specific synapses. (Reproduced, with permission, from Sanes and Yamagata 2009.)
A. The dendrites of retinal ganglion neurons receive input from the processes of retinal interneurons (amacrine and bipolar cells) in the inner plexiform layer, which is subdivided into at least 10 sublaminae. Specific subsets of interneurons and ganglion cells often arborize and synapse in just one layer. These lamina-specific connections determine which aspects of visual stimuli (their onset or offset) activate each type of retinal ganglion cell. The responses of OFF and ON retinal ganglion cells are shown on the right.
B. Immunoglobulin superfamily adhesion molecules (Sdk1, Sdk2, Dscam, and DscamL) are expressed by different subsets of amacrine and retinal ganglion neurons in the developing chick embryo. Amacrine neurons that express one of these four proteins form synapses with retinal ganglion cells that express the same protein. Manipulating Sdk or Dscam expression alters these patterns of lamina-specific arborization.
One clue to the establishment of lamina-specific synapses in the retina comes from the finding that subsets of ganglion neurons in the chick embryo express different classes of immunoglobulin-like adhesion molecules (see Chapter 54). These proteins promote homophilic interactions, that is, they bind to the same protein on other cell surfaces. Moreover, the amacrine and bipolar cells that contact a particular ganglion cell type in a specific sublamina often express the same adhesion molecules as their target cell (Figure 55-2B). When the characteristic adhesion molecule of a chick retinal ganglion neuron is removed, the cell’s dendrites are no longer confined to a specific lamina. Conversely, the dendrites shift to a new lamina when they are forced to express a new immunoglobulin adhesion molecule. Thus a “like-binds-like” recognition system appears to contribute to this instance of synaptic specificity.
A different type of specificity is evident in the olfactory system. In the olfactory epithelium each neuron expresses just one of approximately 1,000 types of receptors. Neurons expressing one receptor are randomly distributed across a large sector of the epithelium, yet all of their axons converge on the dendrites of just a few target neurons in the olfactory bulb, forming synapse-rich glomeruli (Figure 55-3A). When an individual olfactory receptor is deleted, the axons that normally express the receptor reach the olfactory bulb but fail to converge into specific glomeruli or to terminate on the appropriate postsynaptic cells (Figure 55-3B). Conversely, when neurons are forced to express a different odorant receptor, their axons form glomeruli at a different position within the olfactory bulb (Figure 55-3C).
Figure 55-3 Olfactory receptors influence the targeting of sensory axons to discrete glomeruli in the olfactory bulb. (Adapted, with permission, from Sanes and Yamagata 2009.)
A. Each olfactory receptor neuron expresses one of approximately 1,000 possible odorant receptors. Neurons expressing the same receptor are distributed sparsely throughout the olfactory epithelium of the nose. The axons of these neurons form synapses with target neurons in a single glomerulus in the olfactory bulb.
B. In mouse mutants in which an odorant receptor gene has been deleted, the olfactory neurons that would have expressed the gene send their axons to other glomeruli, in part because these neurons now express other receptors.
C. When one odorant receptor gene replaces another in a set of olfactory sensory neurons, their axons project to a new glomerulus. Odorant receptor expression may set the overall activity level of the neuron, thus influencing the nature and level of expression of axonal guidance and recognition molecules.)
Together these experiments suggest that olfactory receptors not only determine a neuron’s responsiveness to specific odorants but also help the axon to form appropriate synapses on target neurons. Specific olfactory receptors may serve as recognition molecules, but it seems more likely that they influence the expression and activity of other receptor systems that contribute to the guidance of olfactory axons. In support of this view, recognition molecules have been found to regulate the interactions of olfactory axons with each other and with their targets.
Different Synaptic Inputs Are Directed to Discrete Domains of the Postsynaptic Cell
Nerve terminals not only discriminate among candidate targets, they also terminate on a specific portion of the target neuron. In many areas of the brain axons arriving in layered structures often confine their terminals to one layer, even if the dendritic tree of the postsynaptic cell traverses numerous layers. In the cerebellum the axons of different types of neurons terminate on distinct domains of the Purkinje neurons. Granule cell axons contact distal dendritic spines, climbing fiber axons contact proximal dendritic shafts, and basket cell axons contact the axon hillock and initial segment (Figure 55-4).
Figure 55-4 The axons of inhibitory inter-neurons in the cerebellum terminate on a distinct region of the cerebellar Purkinje cell. Many neurons form synapses on cerebellar Purkinje neurons, each selecting a distinct domain on the Purkinje cell. The axons of inhibitory basket cells form most of their synapses on the axon hillock and initial segment. Basket cells select these domains by recognizing neurofascin, a cell surface immunoglobulin superfamily adhesion molecule that is anchored to the initial segment of the axon by ankyrin G. When the localization of neurofascin is perturbed, basket cell axons fail to restrict synapse formation to the initial segment. (Adapted, with permission, from Huang 2006.)
Such specificity presumably relies on molecular cues on the postsynaptic cell surface. For Purkinje neurons of the cerebellum one such cue is neurofascin, an adhesion molecule of the immunoglobulin superfamily. Neurofascin is present at high levels on the axonal initial segment and directs basket cells to form axons selectively on this axonal domain. Adhesion molecules can therefore also serve as recognition molecules for particular domains of a neuron. Since individual neurons can form synapses with several classes of pre- and postsynaptic cells, it follows that each neuronal subtype must express a variety of synaptic recognition molecules.
Neural Activity Sharpens Synaptic Specificity
So far we have emphasized the role of recognition molecules in the formation of synapses. Once synapses form, neural activity within the circuit plays a critical role in refining synaptic patterns. In the retinotectal system interactions between ephrins and Eph kinases result in formation of a crude retinotopic map in the tectum (see Chapter 54).
But activity-dependent processes refine the axonal arbors of retinal ganglion cells, thus sharpening the tectal map (Figure 55-5). The axons of retinal ganglion neurons initially form broad diffuse arbors, which gradually become denser but more focused. This refinement is inhibited when the activity of synapses is blocked. Likewise, dendritic arbors of some retinal ganglion cells initially span multiple sublaminae in the inner plexiform layer, then become restricted to narrow ones. The molecular mechanisms of this activity-dependent refinement are largely unknown. One idea is that the level and pattern of neuronal activity regulates the expression of recognition molecules.
Figure 55-5 Electrical activity refines the specificity of synaptic connections in the retina. Some retinal ganglion cells initially form dendritic arbors that are limited to specific sublaminae in the inner plexiform layer of the retina, whereas others initially form diffuse arbors that are later pruned to form large specific patterns. Similarly, the axonal arbors of retinal ganglion cells initially innervate a large region of their target fields in the lateral geniculate nucleus and optic tectum. This expansive axonal arbor is then refined so as to concentrate many branches in a small region. Abolishing electrical activity in retinal ganglion cells decreases the remodeling of dendritic and axonal arbors.
These examples illustrate a widespread phenomenon: Molecular cues control initial specificity but, once the circuit begins to function, specificity is sharpened through neural activity. In the two cases mentioned here, sharpening involves loss of synapses. We will return to the process of synapse elimination at the end of this chapter, and consider its consequences for behavior in the next chapter.
In a few cases neural activity can turn an inappropriate target into an appropriate one. This mechanism has been most clearly demonstrated in skeletal muscle, where mammalian muscle fibers can be divided into several categories according to their contractile characteristics. Muscle fibers of particular types express genes for distinctive isoforms of the main contractile proteins, such as myosins and troponins.
Few muscles are composed exclusively of a single type of fiber; most have fibers of all types. Yet the branches of an individual motor axon innervate muscle fibers of a single type, even in “mixed” muscles in which fibers of different types are intermingled (Figure 55-6A). This pattern, sometimes termed motor unit homogeneity, implies a remarkable degree of synaptic specificity. However, matching does not come about solely through recognition in the motor axon of the appropriate type of muscle fiber. The motor axon can also convert the target muscle fiber to an appropriate type. When a muscle is deinnervated at birth, before the properties of its fibers are fixed, a nerve that normally innervates a slow muscle can be redirected to reinnervate a muscle destined to become fast, and vice versa. Under these conditions the contractile properties of the muscle are partially transformed in a direction imposed by the firing properties of the motor nerve (Figure 55-6B, C).
Figure 55-6 The pattern of motor neuron activity can change the biochemical and functional properties of skeletal muscle cells.
A. Muscle fibers have characteristic metabolic, molecular, and electrical properties that identify them as “slow” (tonic) or “fast” (phasic) types. The micrograph on the right shows a section of muscle tissue with histochemical staining for myosin ATPase. The middle sketch shows a section through the muscle, in which motor neurons (green and brown) form synapses on a single type of muscle fiber. (Photo reproduced, with permission, from Arthur P. Hays.)
B. Motor neurons that connect with fast and slow muscle fibers (fast and slow motor neurons) exhibit distinct patterns of electrical activity: steady low frequency (tonic) for slow and intermittent high-frequency bursts (phasic) for fast.
C. Cross-innervation experiments showed that some property of the motor neuron helps to determine whether muscle fibers are fast or slow. Cross-innervation was achieved by surgically rerouting fast axons to slow muscle and vice versa. Although the properties of the motor neurons are little changed, the properties of the muscle change profoundly. For example, fast motor neurons induce fast properties in the slow muscle. (Adapted, with permission, from Salmons and Sreter 1976.)
D. The effects of innervation by fast and slow nerves on muscle are mediated in part by their distinct patterns of activity. When a fast muscle is stimulated in a slow tonic pattern, the muscle acquires slow electrical and molecular properties. Conversely, fast phasic stimulation of a once slow muscle can convert it to a faster type.
Different patterns of neural activity are responsible for the switch in muscle properties. Most strikingly, direct electrical stimulation of a muscle with patterns normally evoked by slow or fast nerves leads to changes that are nearly as dramatic as those produced by cross-innervation (Figure 55-6D). Although activity-based conversion of the type used at the neuromuscular junction is unlikely to be a major contributor to synaptic specificity in the central nervous system, it raises the possibility that central axons can modify the properties of their synaptic targets, contributing to the diversification of neuronal subtypes and refining connectivity imposed by recognition molecules.
Principles of Synaptic Differentiation Are Revealed at the Neuromuscular Junction
The neuromuscular junction comprises three types of cells: a motor neuron, a muscle fiber, and Schwann cells. All three types are highly differentiated in the region of the synapse.
The process of synapse formation is initiated when a motor axon, guided by the multiple factors described in Chapter 54, reaches a developing skeletal muscle and approaches an immature muscle fiber. Contact is made and the process of synaptic differentiation begins. As the growth cone begins its transformation into a nerve terminal, the portion of the muscle surface opposite the nerve terminal begins to acquire its own specializations. As development proceeds, synaptic components are added and structural signs of synaptic differentiation become apparent in the pre- and postsynaptic cells and in the synaptic cleft. Eventually the neuromuscular junction acquires its mature and complex form (Figure 55-7A, B).
Figure 55-7 The neuromuscular junction develops in sequential stages.
A. A growth cone approaches a newly fused myotube (1) and forms a morphologically unspecialized but functional contact (2). The nerve terminal accumulates synaptic vesicles and a basal lamina forms in the synaptic cleft (3). As the muscle matures, multiple axons converge on a single site (4). Finally, all axons but one are eliminated and the surviving terminal matures (5). (Adapted, with permission, from Hall and Sanes 1993.)
B. At the mature neuromuscular junction, pre- and postsynaptic membranes are separated by a synaptic cleft that contains basal lamina and extracellular matrix proteins. Vesicles are clustered at presynaptic release sites, transmitter receptors are clustered in the postsynaptic membrane, and nerve terminals are coated by Schwann cell processes. (Image reproduced, with permission, courtesy of T. Gillingwater.)
Three general features of neuromuscular junction development have provided clues about the molecular mechanisms that underlie synapse formation. First, nerve and muscle organize each other’s differentiation. In principle, the precise apposition of pre- and postsynaptic specializations might be explained by independent programming of nerve and muscle properties. However, in muscle cells cultured alone acetylcholine (ACh) receptors are generally distributed uniformly on the surface, although some are clustered as in mature postsynaptic membranes. Yet when motor neurons are added to the cultures they extend neurites that contact the muscle cells more or less randomly, instead of seeking out the ACh receptor clusters. New receptor clusters appear precisely at the points of contact with the presynaptic neurites while preexisting uninnervated clusters eventually disperse (Figure 55-8). Thus factors on or released by motor axons exert a profound influence on the synaptic organization of the muscle cell. Likewise, muscles signal retrogradely to motor nerve terminals. When motor neurons in culture extend neurites, they assemble and transport synaptic vesicles, some of which form aggregates similar to those found in nerve terminals. When the neurites contact muscle cells, new vesicle clusters form opposite the muscle membrane, and most of the preexisting clusters disperse.
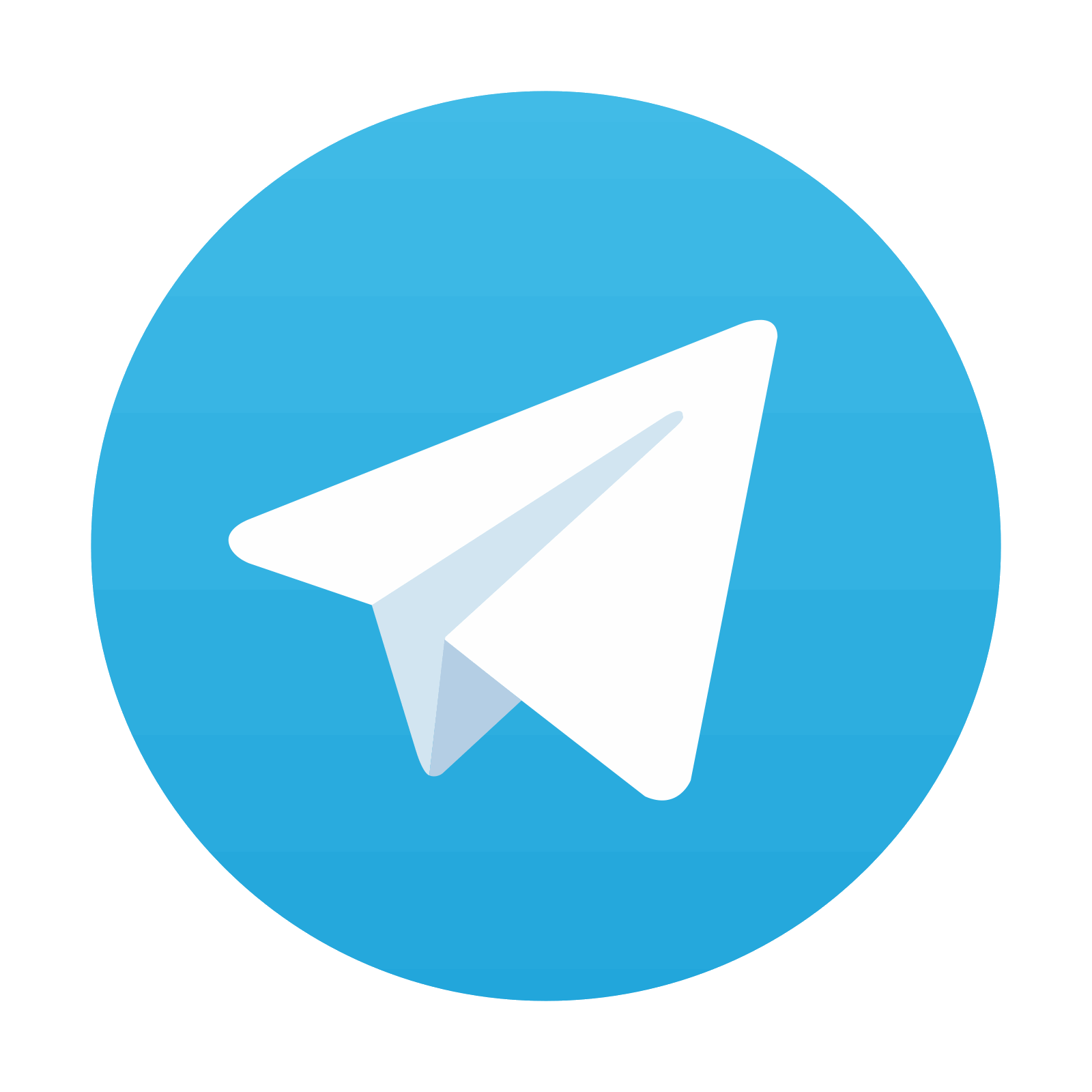
Stay updated, free articles. Join our Telegram channel
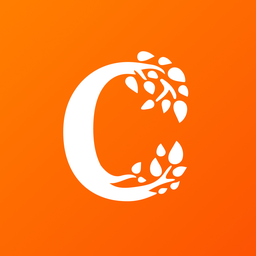
Full access? Get Clinical Tree
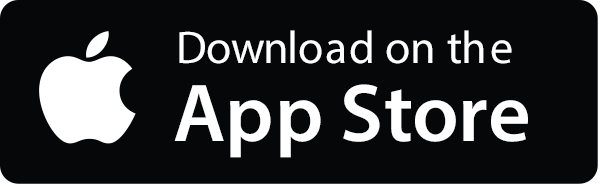
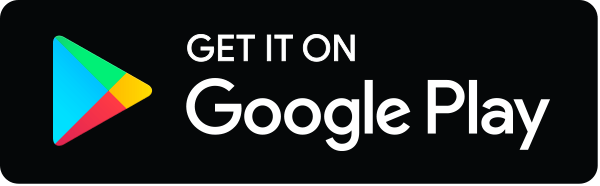