From Nerve Cells to Cognition: The Internal Representations of Space and Action
The Brain Has an Orderly Representation of Personal Space
The Cortex Has a Map of the Sensory Receptive Surface for Each Sensory Modality
Cortical Maps of the Body Are the Basis of Accurate Clinical Neurological Examinations
The Internal Representation of Personal Space Can Be Modified by Experience
Extrapersonal Space Is Represented in the Posterior Parietal Association Cortex
Much of Mental Processing Is Unconscious
Is Consciousness Accessible to Neurobiological Analysis?
Consciousness Poses Fundamental Problems for a Biological Theory of Mind
Selective Attention to Visual Stimuli Can Be Studied on the Cellular Level in Nonhuman Primates
NEURAL SCIENTISTS BELIEVE THAT a cellular approach is necessary to understand how the brain works. Considering that the brain has a hundred billion nerve cells, it is remarkable how much can be learned about mental activity by examining one nerve cell at a time. Progress is particularly good when we understand the anatomy and connections of functionally important pathways.
Cellular studies of the sensory systems, for example, provide important insight into how stimuli at the body’s surface are translated by the brain into sensations and planned action. In the visual system, the sensory system most thoroughly studied at the cellular level, information arrives in the brain from the retina in parallel pathways dedicated to analyzing different aspects of the visual image—form, movement, and color. These separate inputs are eventually integrated into coherent images according to the brain’s own rules, rules that are embodied in the circuitry of the visual system.
Different modalities of perception—an object seen, a face touched, or a melody heard—are processed similarly by the different sensory systems. Receptors in each system first analyze and deconstruct stimulus information. Receptors at the periphery of the body for each system are sensitive to a particular kind of physical event—light, pressure, tone, or chemical odorants. When a receptor is stimulated—when, for example, a receptor cell in the retina is excited by light—it responds with a distinct pattern of firing that represents certain properties of the stimulus. Each sensory system obtains information about the stimulus in this way and this information is conveyed along a pathway of cells leading to a specific (unimodal) region of cerebral cortex. In the cortex different unimodal regions representing different sensory modalities communicate with multimodal association areas through specific intracortical pathways, and in this network signals are selected and combined into an apparently seamless perception.
The brain thus produces an integrated perception because nerve cells are wired together in precise and orderly ways according to a general plan that does not vary greatly among normal individuals. Nevertheless, the connections are not exactly the same in all individuals. As we shall learn in later chapters, connections between cells can be altered by activity and by learning. We remember specific events because the structure and function of the connections between nerve cells are modified by those events.
Despite its success, neural scientists believe that a cellular approach alone is not sufficient for understanding how the integrative action of the brain—the simultaneous activity of discrete sets of neurons—produces cognition. For this task the brain must be studied as an information processing organ. This is the approach of cognitive neural science, which uses a combination of methods from a variety of fields—cell biology, systems neural science, brain imaging, cognitive psychology, behavioral neurology, and computational neuroscience.
Ulric Neisser, one of the pioneers of cognitive psychology, defined the challenge of this field in the following terms:
It has been said that beauty is in the eye of the beholder. As a hypothesis … it points clearly enough to the central problem of cognition—the world of experience is produced by the man who experiences it …. There certainly is a real world of trees and people and cars and even books, and it has a great deal to do with our experience of these objects. However, we have no direct immediate access to the world, nor to any of its properties….
Whatever we know about reality has been mediated not only by the organs of sense but by complex systems which interpret and reinterpret sensory information…. The term “cognition” refers to all the processes by which the sensory input is transformed, reduced, elaborated, stored, recovered and used….
In this chapter we first discuss how cognitive neural science evolved from otherwise disparate disciplines. We illustrate the success of the approach by considering what has been learned about one complex mental state: the experience of personal and extrapersonal space, both real and imagined. We then discuss how the unconscious and conscious mental processes are modeled by cognitive neural science. A great deal of cognitive processing goes on unconsciously. Sigmund Freud likened the conscious perception of mental processes to the perception of the external world by sense organs. We also discuss the profound challenges to a scientific study of consciousness. The five major subjects of cognitive neural science—perception, action, emotion, language, and memory—are discussed in detail in the subsequent five parts of the book beginning with Chapter 21.
The Major Goal of Cognitive Neural Science Is to Understand Neural Representations of Mental Processes
Until the end of the 19th century the chief method for understanding the mind was introspection. In fact, the scholarly study of the mind was a branch of philosophy. By the middle of the 19th century, however, the philosophical approach was giving way to empirical analysis and eventually the formation of the independent discipline of experimental psychology. In its early years experimental psychology was concerned primarily with the sequence of events by which an external stimulus becomes an internal sensation. By the end of the 19th century the interests of psychologists turned to how behavior is generated, how it is modified by learning and attention, and how it is stored in memory.
The discovery of simple experimental ways of studying learning and memory—first in human beings by Hermann Ebbinghaus in 1885 and a few years later in experimental animals by Ivan Pavlov and Edgar Thorndike—led to a rigorous empirical school of psychology called behaviorism. Behaviorists, notably J. B. Watson and B. F. Skinner in the United States, argued that behavior could be studied with the precision of the physical sciences, but only if psychologists abandoned speculation about what occurs in the mind and focused exclusively on the observable aspects of behavior. For example, the behaviorists argued that one cannot base a psychology on the idea that people do certain things because they believe they are the right things to do or because they want to do them.
Behaviorists regarded these unobservable mental processes, especially anything as abstract as motivations, feeling, or conscious awareness, as inaccessible to scientific study. They concentrated instead on evaluating—objectively and precisely—the relationship between specific physical stimuli and observable responses in intact animals. Their early successes in studying simple forms of behavior and learning encouraged them to treat all cognitive processes that intervene between the stimulus (input) and behavior (output) as irrelevant.
During behaviorism’s most influential period, in the 1950s, many psychologists accepted the most radical behaviorist position: that observable behavior is all there is to mental life. As a result, the scientific concept of behavior largely depended on the techniques used to study it. This emphasis reduced the domain of experimental psychology to a restricted set of problems and excluded some of the most fascinating features of mental life.
By the 1960s it was not difficult for the founders of cognitive psychology—notably Edwin Tolman, Frederick Bartlett, George Miller, Noam Chomsky, Ulric Neisser, and Herbert Simon—to convince the scientific community that behaviorism was too limiting Building on earlier evidence from Gestalt psychology, psychoanalysis, and neurology, these early cognitive psychologists sought to demonstrate that our knowledge of the world is based on our biological equipment for perceiving the world, that perception is a constructive process that depends not only on the stimulus but also on the mental apparatus of the perceiver—the organization of the sensory and motor systems in the brain. We now realize that this constructive process also involves emotion, motivation, and reward.
What ultimately distinguished the cognitivists from the behaviorists was not only their conceptual approach to behavior but also the complexity of the methods they used. Cognitivists realized that only relatively few input–output relationships are stereotyped, that these relationships vary significantly because of mental states, past history, and expectations, the very factors that the behaviorists tended to ignore. Thus these variables must also be observable in behavior (or output) but are just more difficult to identify than the behavior defined by behaviorists.
This new perspective relied on neural network modeling and fortunately it coincided with the emergence of large-scale computers in the period following World War II. Computers allowed the modeling and testing of ideas about large neural networks that in principle are capable of higher mental functions. However, once psychologists acknowledged that mental activity was equivalent to computational processes in the brain, they had to face the fact that most mental processes were still largely inaccessible in living subjects. Without direct access to the brain, it would be difficult if not impossible to choose between various rival theories.
Fortunately, new tools for the empirical study of mental processes quickly became available, and significant progress was soon made in cellular analyses of the neural mediation of vision, touch, and action in intact primates engaged in ordinary behavior. Singleneuron recording and noninvasive imaging and recording techniques have allowed researchers to describe how neural activity in different sensory and motor pathways encodes sensory stimuli and planned actions. Moreover, imaging methods permit direct visualization of the brain in human subjects engaged in mental activity, allowing insight into attention and aspects of consciousness under controlled conditions (Chapter 20). Thus we can now study directly neural representations of the environment and motor action by comparing cellular recordings in primates engaged in purposeful activity with imaging of the human brain at work.
Cognitive neural science, as now practiced, emerged from four major technical and conceptual developments. First, in the 1960s and 1970s techniques were developed by Robert Wurtz and Edward Evarts at the National Institutes of Health for studying the activity of single cells in the brains of animals, including primates, engaged in controlled behavior in the laboratory. This allowed investigators to correlate the activity of specific populations of neurons with specific perceptual and motor processes. From these microelectrode studies we have been able to see that the mechanisms of perception are much the same in humans, monkeys, and even simpler animals.
These cellular studies in monkeys also made it possible to identify the importance of different combinations of areas of the brain involved in specific cognitive functions, such as attention and decision-making. These approaches changed the way the biology of behavior is studied both in experimental animals and in humans.
Second, developments in neural science and cognitive psychology stimulated a renewed interest in the behavioral analysis of patients with brain lesions that interfere with mental functioning. This area, neuropsychology, had remained a strong subspecialty of neurology in Europe but was neglected for a time in the United States. Lesions of different regions of the brain can result in quite specific cognitive deficits. The behavioral consequences of brain lesions thus tell us much about the function of specific neural pathways. Lesion studies have shown that cognition is the product of several specialized systems, each with many components. For example, the visual system has specialized pathways for processing information about color and form on the one hand and movement on the other.
Third, the development of imaging techniques such as positron emission tomography (PET) and functional magnetic resonance imaging (MRI), as well as the development of magnetoencephalography, has made it possible to relate changes in the activity of large populations of neurons to specific mental acts in living humans (see Chapter 20). This advance has been paralleled by two further developments. The use of voltage and calcium-sensitive dyes has permitted the study of neuronal activity in large ensembles of neurons, both in vitro and in the brains of behaving animals. The more recent use of light-sensitive ion channels has permitted the activation or inactivation of the activity of specific neurons or groups of neurons in the neural circuits of intact behaving animals.
Finally, improvement in computers and the emergence of a powerful subdiscipline of computational neural science has made it possible to model the activity of large populations of neurons and to test ideas about the roles of specific components of neural circuits in the brain in particular behaviors. To understand the neural organization of a complex behavior like speech we must understand not only the properties of individual cells and pathways but also the network properties of circuits in the brain. Although network properties depend on the properties of individual neurons in the network, they are not identical or even similar to those properties but are an emergent property of the way those different cells are interconnected.
When computational approaches are combined with detailed behavioral studies, for example with the psychophysical study of a specific perceptual act, the combined analysis can help to characterize the properties of a system. Thus psychophysics can describe what the system is capable of doing whereas computational modeling can describe how the properties of constituent cells could account for system properties of the neural circuits involved (see Appendices E and F).
The work of cognitive and computational neuro-scientists is providing enormous insight into the workings of the brain. However, it also raises a difficult set of questions about the relationship between observed neurophysiological and mental processes, and particularly between these cellular biological processes and consciousness. The answers to these questions are yet unknown, but the mere fact that scientists are addressing them is a major advance.
To illustrate how cognitive neural science describes a mental act, in the next few sections we summarize what neural science has learned about the brain’s representation of personal space (one’s body) and peripersonal space, the space within arm’s length (“near space”). We shall see how the brain constructs mental representations of space from external sensory input; how this representation gives rise to imagined and remembered space; and how it is selected by action, modified by normal experience, and distorted by abnormal experience such as loss of a body part. This discussion illustrates a key principle that we will consider again in Chapter 19, that action has a key role in perception. The cognitive functions of the premotor areas provide for flexibility in behavior, preventing an otherwise stereotypic relationship between sensory input and behavioral output. By interpreting sensory input based on experience and mental state, these cognitive premotor processes shape our behavior.
The neural representation of space is most clearly evident in the early stages of sensory processing—in primary and higher-order areas of somatosensory cortex—where it takes the form of a map of the tactile sensors on the body surface. We shall see how this map can be modified after the loss of a body part and how those modifications can create a phantom representation. We shall also see how representations of personal and peripersonal space differ from extrapersonal space, the space beyond arm’s length (“far space”), and how representations of extrapersonal space can give rise to imagined and remembered space.
The Brain Has an Orderly Representation of Personal Space
The neural representation of the body surface is a simple example of an internal representation, one that has been extensively explored in the study of touch and proprioception. Touch provides information about the properties of objects, such as their shape, texture, and solidity; proprioception provides information about the static position and movement of fingers and limbs.
An internal representation can be thought of as a certain pattern of neural activity that has at least two aspects: (1) the pattern of activation within a particular population of neurons (some cells are active and others not) and (2) the pattern of firing in individual cells.
Sensory neurons with receptors in the skin translate the mechanical energy of a stimulus into neural signals that are then conveyed along pathways that end in the somatosensory areas of the parietal lobe of the cerebral cortex (Figure 17-1). Each pathway includes one or more synaptic relays. At each relay, where thousands of afferent axons terminate on a cluster of similar neurons, the arrangement of the presynaptic fibers preserves the spatial relations of the receptors on the body surface. This somatotropic order thus creates a neural map of the body surface at each synaptic relay in the somatosensory system—information from neighboring receptors in the skin is conveyed to neighboring cells in each synaptic relay.
Figure 17-1 The somatosensory system in the cerebral cortex. A lateral view of a cerebral hemisphere illustrates the location of the primary somatic sensory cortex in the parietal lobe. The somatic sensory cortex has three major divisions: the primary (S-I) and secondary (S-II) somatosensory cortices and the posterior parietal cortex. A sagittal section shows the distinct cytoarchitec-tonic regions of S-I (Brodmann’s areas 3a, 3b, 1, and 2) and the adjacent posterior parietal cortex (areas 5 and 7) and motor cortex (area 4).
Neural maps of the body surface were first detected in laboratory animals using gross recording and stimulation techniques on the surface of the postcentral gyrus of the parietal cortex, the only portion of the cortex accessible with the experimental techniques available at that time. In the late 1930s Wade Marshall found that he could produce an evoked potential in the cortex by touching a specific part of the animal’s body surface (Figure 17-2). Evoked potentials are electrical signals that represent the summed activity of thousands of cells and are recorded with external macroelectrodes on the brain surface. The evoked response method was used by Marshall, Clinton Woolsey, and Phillip Bard to map the neural representation of the body surface in the postcentral gyrus of monkeys (Figure 17-3).
Figure 17-2 Evoked potentials in the somatosensory cortex elicited by stimulation of the hand. The evoked potentials shown here are the summed activity of one large group of neurons in the left postcentral gyrus of a monkey, elicited by a light touch at different points on the right palm. The evoked potentials were strongest when the thumb or forefinger was stimulated (points 15, 16, 17, 20, 21, and 23) and weakest when the middle or small finger was stimulated (points 1, 2, 3, 12, and 13). (Adapted, with permission, from Marshall, Woolsey, and Bard 1941.)
Figure 17-3 An early map of the representation of the hands in the monkey cortex. Recordings were made in the primary somatic sensory cortex (S-I) in the postcentral gyrus. The lateral view of the brain shows the recording site. Sites in Brodmann’s areas 3b and 1 that responded to stimulation of the palmar and dorsal surfaces of the right hand are identified by black dots in a schematic map of these areas. The area of the hand that evoked a response at each site is indicated by the colored portion of the hand. The sites in the anterior wall of the postcentral gyrus are roughly in areas 3b and 3a (see Figure 17-1). The sites on the dorsal surface of the postcentral gyrus are roughly in area 1. (Adapted, with permission, from Marshall, Woolsey, and Bard 1941.)
The human somatosensory cortex was similarly mapped in the late 1940s by the Canadian neurosurgeon Wilder Penfield during operations for epilepsy and other brain disorders. Working with locally anesthetized conscious patients, Penfield stimulated various points on the surface of the postcentral gyrus and asked the patients to report what they felt. This procedure was necessary to ascertain where the epilepsy started and therefore to avoid damage to healthy brain tissue during surgery. Penfield found that activation of specific populations of cells in the postcentral gyrus reasonably simulated natural activation of these populations, producing sensations of touch and pressure in the contralateral hand or leg. From these studies Penfield constructed the neural map of the human body in the primary somatosensory cortex.
In this map the leg is represented in the most medial area of cortex followed by the trunk, arms, face, and finally, most laterally, the teeth, tongue, and esophagus. The area devoted to each part of the body reflects the relative importance of that part in sensory perception. Thus the area for the face is large compared with that of the back of the head, that of the index finger is gigantic compared with the big toe, and the torso is represented in the smallest area of all (Figure 17-4). Such differences reflect differences in innervation density throughout the body. Similar relationships are observed in other animals. In rabbits, for example, the face and snout have the largest cortical representation because they are the most important sensory surfaces a rabbit uses to explore its environment (Figure 17-5).
Figure 17-4 Cortical representations of the parts of the body correspond to the sensory importance of each part. Each of the four areas of the somatosensory cortex forms its own map of the body (see Figure 17-6). The sensory homunculus illustrated here is based on the body map in area 1 in the postcentral gyrus. This area receives inputs from tactile receptors in the skin throughout the body. Areas of cortex representing parts of the body that are especially important for tactile discrimination, such as the tip of the tongue, fingers, and hand, are disproportionately large, reflecting the greater degree of innervation in these parts. (Adapted, with permission, from Penfield and Rasmussen 1950.)
Figure 17-5 Cortical somatosensory maps in different species reflect different somatic sensibilities. These drawings show the relative importance of body regions in the somatosensory cortex of four species, based on evoked potentials in the thalamus and cortex.
The Cortex Has a Map of the Sensory Receptive Surface for Each Sensory Modality
Marshall went on to find that the receptive surfaces for vision and hearing, the retina and the cochlea, are also represented topographically in the cortex. Marshall’s early efforts to analyze these sensory maps of the body probed only limited areas of the cortex and used techniques with poor spatial resolution. His work in the area of touch led to the conclusion that there is a single large representation of the body surface in the cerebral cortex. Later studies based on single-neuron recordings revealed four fairly complete maps in the four areas of the primary somatosensory cortex (Figure 17-6).
Figure 17-6 Each of the four areas of the primary somatic sensory cortex forms its own complete representation of the body surface. (Adapted with permission, from Kaas et al. 1981.)
A. Somatosensory maps of the body in Brodmann’s areas 3b and 1 are shown in this dorsolateral view of the brain of an owl monkey. The two maps are roughly mirror images. Each digit of the hands and feet is individually represented (D1 to D5). Areas 2 and 3a (not shown) have a similar organization.
B. This more detailed illustration of the representation of the palm in areas 3b and 1 shows discrete areas of representation of the palmar pads (P4 to P1), two insular pads (I), two hypothenar pads (H), and two thenar pads (T).
C. This idealized representation of the hands in the somatosensory cortex is based on studies of a large number of monkeys. The areas of cortex devoted to the palm and digits reflect the extent of innervation of each part of the hand. The five digital pads (D1 to D5) include distal, middle, and proximal segments (d, m, p).
Although each of the four areas has essentially the same body map, each area processes a distinct type of information. Area 3a receives information from muscles and joints, important for limb proprioception. Area 3b receives information from the skin, important for touch. This information from the skin is further processed within area 1 and then combined with information from muscles and joints in area 2. This explains why a small lesion in area 1 impairs tactile discrimination, whereas a small lesion in area 2 impairs the ability to recognize the shape of a grasped object.
Cortical Maps of the Body Are the Basis of Accurate Clinical Neurological Examinations
The existence in the brain of maps of the sensory receptive surface and a similar motor map for movement explains why clinical neurology can be an accurate diagnostic discipline, even though for many decades before brain imaging came along neurology relied on only the simplest tools: a wad of cotton, a safety pin, a tuning fork, and a rubber reflex hammer. For example, disturbances in the somatic sensory system can be located with remarkable accuracy because there is a direct relationship between the anatomical organization of the functional pathways in the brain and specific perceptual and motor behaviors.
A dramatic example of this relationship is the Jacksonian march, a characteristic sensory seizure first described by the British neurologist John Hughlings Jackson. In this type of seizure there is, in addition to a motor progression, a sensory progression. Numbness and paresthesia (inappropriate sensations such as burning or prickling) begin in one place and spread throughout the body. For example, numbness might start at the fingertips, spread to the hand, up the arm, across the shoulder into the back, and down the leg on the same side. This sequence is explained by the arrangement of inputs from the body in the somatosensory cortex (Figure 17-4); the seizure starts in the lateral region of the cortex, in the area where the hand is represented, and propagates across the cortex toward the midline.
The Internal Representation of Personal Space Can Be Modified by Experience
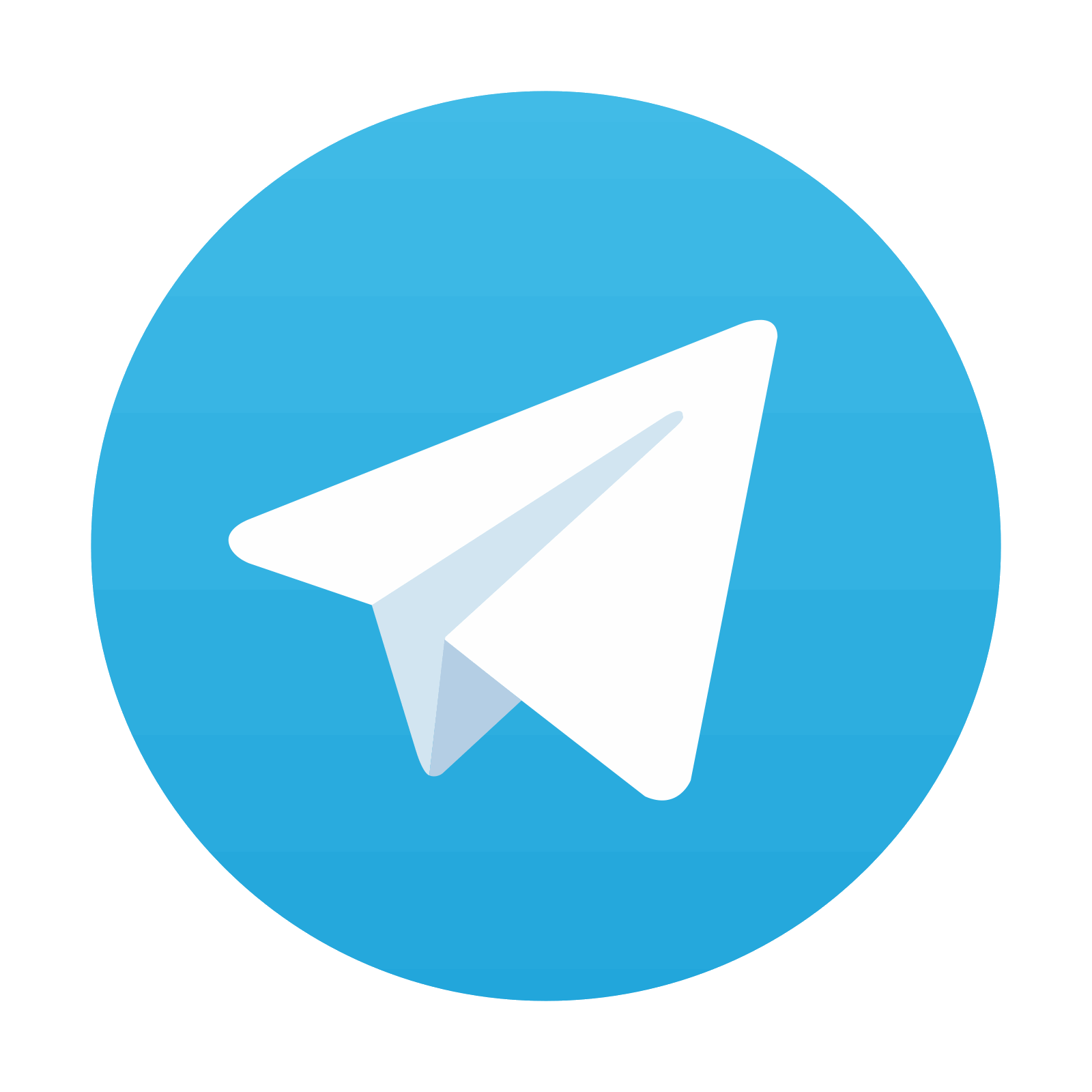
Stay updated, free articles. Join our Telegram channel
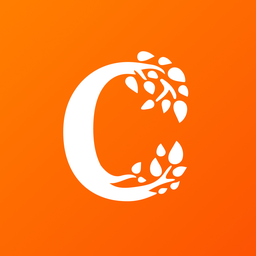
Full access? Get Clinical Tree
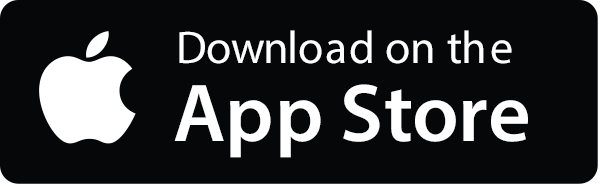
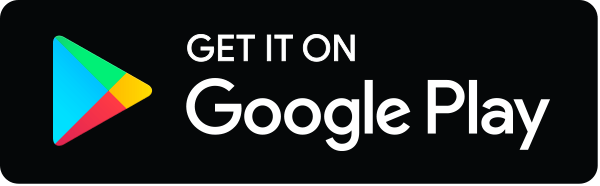