© Springer Science+Business Media New York 2016
Fredric P. Manfredsson (ed.)Gene Therapy for Neurological DisordersMethods in Molecular Biology138210.1007/978-1-4939-3271-9_2727. Gene Therapy-Based Modeling of Neurodegenerative Disorders: Huntington’s Disease
(1)
Department of Pharmacology & Clinical Pharmacology & Centre for Brain Research, School of Medical Sciences, University of Auckland, Auckland, New Zealand
Abstract
Huntington’s disease is a fatal neurodegenerative disease characterized by impairments in motor control, and cognitive and psychiatric disturbances. In this chapter, viral vector-mediated approaches used in modeling the key neuropathological features of the disease including the production of abnormal intracellular protein aggregates, neuronal dysfunction and degeneration and motor impairments in rodents are described.
Key words
Viral vector Huntingtin Transgenic Genetic Neurons Neurodegenerative disease 1 Introduction
Huntington’s disease (HD) is a progressive and devastating neurodegenerative disorder characterized by a triad of symptoms including motor dysfunction, cognitive decline, and psychiatric disturbances. There is currently no effective treatment for HD, a disease that progresses towards death within approximately 20 years of disease onset [1]. HD is inherited in an autosomal dominant manner, with the underlying genetic mutation being an expansion of a CAG repeat sequence in exon 1 of huntingtin (HTT). This results in the production of huntingtin protein (HTT) with an expanded polyglutamine (polyQ) tract. Expansion to greater than 36 CAG repeats results in the disease, with increased tract lengths associated with an earlier disease onset. Current hypotheses posit that HD could be caused by the dominant-negative action of mutant HTT resulting in a loss of function of the wild-type protein, but increasing evidence suggests a toxic gain of function of mutant HTT (mHTT) is more likely to be causative of HD pathogenesis. The key neuropathological hallmarks of HD are abnormal intracellular aggregates composed of mHTT and other sequestered proteins including transcription factors, neuronal dysfunction and neurodegeneration [2]. The brain regions most profoundly affected are the striatum, where extensive loss of GABAergic projection neurons results in significant atrophy of the striatum. Neuronal loss resulting in the thinning of the cortical layers is also found [3], with the hippocampus, hypothalamus, and substantia nigra also affected [4].
Various animal model s of HD have been developed that reproduce specific neuropathological features of the disease. There is no single model that fulfills the criteria of modeling all aspects of the human disease, with each type of model having specific strengths but also limitations [5]. Early studies utilized specific neurotoxins that induce excitotoxicity (glutamate-, kainic acid-, quinolinic acid) [6–9] or impair mitochondrial function (3-nitroprionic acid, malonate [10–12]) to produce the characteristic degeneration of neurons in the striatum in rodents and primates. These models have largely been superseded by genetic approaches that aim to emulate the molecular pathogenic mechanisms underlying HD. Transgenic animal models including C. elegans [13, 14], Drosophila [15–17] and mice that express full-length, N-terminal fragments of mHTT or knock-in of mHTT sequences into the endogenous Htt locus of the animal have been developed [18–22]. Specific hallmarks of the human disease are reproduced including nuclear and neuritic aggregates that are ubiquitinated, neuronal dysfunction and specific impairments in motor function. Of note, although striatal atrophy and neuronal cell death has been reported in several mouse models [23–25], no striatal neuronal cell death is found in other transgenic rodent models [26, 27].
Viral vector -based modeling of HD offers several advantages over traditional transgenic approaches. Expression cassettes varying in truncated N-terminal fragments and CAG repeat length under the control of regulatable elements or cell or tissue-specific promoters that direct higher levels of transgene expression than can be achieved in transgenic mouse models can be rapidly developed. Expression cassette design coupled with the engineering of viral capsids can be exploited to direct transgene expression to neurons or astrocytes. Viral vectors can also be injected at any developmental age in a broad range of species including mice, rats, and nonhuman primates, making it a very versatile tool for disease modeling purposes. Lentiviral, adenoviral, and adeno-associated viral (AAV ) vectors have been exploited as gene transfer agents for HD modeling studies both in an in vitro and in vivo setting as discussed below.
1.1 Using Viral Vectors to Model HD In Vitro
One advantage of many viral vectors is their ability to efficiently transduce primary neuronal cultures, facilitating studies on the molecular mechanisms underlying mHTT neuropathology. Primary striatal neurons infected with lentiviral vectors expressing N-terminal mHTT expressing 82 polyQ (mHTT82Q) under control of the mouse phosphoglycerate kinase 1 (PGK) promoter develop nuclear and neuritic aggregates that subsequently lead to neuronal dysfunction and toxicity by 6–8 weeks [28]. Aggregate neuropathology is also observed following transduction of primary cortical neurons but in contrast, no signs of neuronal dysfunction were observed, consistent with the selective vulnerability of GABAergic medium spiny neurons to mHTT. The rate of formation of HTT inclusions is considerably slower in cells and is also found in neuritic and cytoplasmic compartments expressing full-length mHTT; in comparison truncated mHTT is found predominantly in the form of nuclear aggregates [29]. Furthermore, Senut and colleagues [30] showed that AAV -mediated expression of GFP fused to expanded CAG repeat lengths in HEK293 cells led to the rapid appearance of GFP-positive cytoplasmic and nuclear aggregates 16 h after infection suggesting that expanded polyQ tracts alone can mediate pathogenic effects.
1.2 Viral Vector-Based Rodent Models of HD
Viral vector -based rodent models of HD are a powerful complement to the existing chemical and transgenic mouse and rat models. The most common strategy employed is to inject viral vectors expressing truncated HTT transcripts with expanded CAG repeats unilaterally into one striatum, leaving the contralateral side to serve as internal control for subsequent biochemical and molecular analyses. Neuropathological analyses can be compared against control animals that express a matching HTT transcript but with normal repeat length (i.e., <36 CAG). Potential new treatments can be initiated either prior or following vector infusion. Most studies have relied on immunohistochemical methods to examine specific aspects of interest in the model including time-dependent changes in mHTT expression levels and intracellular aggregate formation using anti-HTT or antibodies that specifically detect aggregated HTT (e.g., EM48), protein partners that interact with HTT and the effects of mHTT on specific striatal neuronal populations (e.g., using antibodies to NeuN, calbindin-D28K, DARPP32, choline acetyltransferase (ChAT), parvalbumin, Neuropeptide Y (NPY)). Other features such as reactive gliosis can be examined as well as behavioral testing of treated animals to assess any deficits in motor function.
In general, the neuropathological features reported between the different studies are remarkably consistent, with a rapid onset of pathology following vector infusion that progresses to significant neuronal cell loss in the striatum by 5–12 weeks. The earliest abnormalities are the appearance of intranuclear neuronal aggregates and inclusions that sequester ubiquitin and other proteins, progressing to neuronal cell loss and striatal atrophy and motor impairment, with variations in the time course of neuropathology dependent on mHTT expression levels, HTT fragment length, and CAG repeat length. I will now cover some specific variables that can influence the model.
1.2.1 Viral Vectors and Vector Infusion
Lentiviral [31] and AAV vectors including AAV2 [30], chimeric AAV1/2 [32], and mosaic AAV1/8 [33] vectors have been used to deliver mHTT or polyQ transcripts into the mouse and rat striatum. AAV1/2 and AAV1/8 vectors transduce a large volume of the striatum compared to AAV2, and possibly lentiviral vectors [31], leading to a greater volume of distribution of transgene expression and production of larger striatal lesions. For example, we found that 35 % of the injected striatum was devoid of staining for the neuronal marker calbindin-D28K, DARPP-32, and NeuN by 5 weeks [32]. However, differences in transduction and lesion volumes could also be largely due to differences in the promoters used in the AAV1/2 and AAV1/8 studies compared to the lentiviral vector study; the PGK and CMV promoters were used in a lentiviral context, whereas the strong CMV/chicken beta actin hybrid promoter and neuron-specific enolase (NSE) promoters were used in the AAV studies [32, 33]. Indeed we found that the AAV1/2-mediated mHTT expression levels rose to >100-fold that of endogenous rat Hdh expression at 2 weeks, while Drouet et al. [34], found that mHTT expression levels under control of the PGK promoter in lentiviral vectors was 25-fold higher than endogenous HTT. In a subsequent study, Regulier and colleagues used a tetracycline-regulated lentiviral vectors to express the first 853 amino acids of mHTT. Expression levels were 4- to 5-fold higher than that achieved using the PGK promoter and induced an early pathological onset and exacerbated the HD neuropathology [35]. One advantage of the tetracycline-regulated system is that it allows conditional expression of mHTT by systemic administration of doxycycline, enabling exploration of the relationship between mHTT protein expression and disease progression.
A snapshot of mHTT dosage effects on the kinetics of aggregate formation or toxicity can often be captured by analyses of brain sections from a 2 to 5 week time-point, although the specific timing of these appearance of this pathology will vary depending on vector system and mHTT fragment expressed. Typically the highest levels of transgene are detected 1–2 mm from the viral vector injection site, and so the earliest appearance of intranuclear inclusions and neuronal depletion occurs in this region. Surrounding the core region of neuronal depletion, neurons express lower levels of transgene expression presumably reflective of the extent of diffusion of vector from the infusion site. These neurons may show evidence of intracellular aggregates.
In humans with HD, there is preferential loss of GABAergic medium spiny neurons. The demonstration that lentiviral-mediated expression of mHTT leads to selective depletion of DARPP32-positive medium spiny neurons but interneurons in the mHTT-injected rats are largely spared is consistent with that observed in human HD [31]. In contrast, we found that the chimeric AAV1/2 vector used in our study transduced striatal medium spiny neurons but there was increased transduction of cholinergic interneurons compared to other neuronal subtypes. This resulted in toxicity and neuronal death of this interneuron population irrespective of the transgene expressed. In contrast, we found that parvalbumin and NPY-positive interneurons were only susceptible to mHTT [32].
Retrograde and anterograde transport of AAV vectors from the striatal injection site can lead to transgene expression and the appearance of intracellular aggregates in distal basal ganglia regions including the substantia nigra (SN) and globus pallidus [30, 32], brain regions where neurodegeneration and atrophy are also observed in humans with HD [36]. We found axonal transport of a chimeric AAV1/2 vector expressing exon 1 of HTT with 70Q was associated neuronal cell loss and atrophy of the globus pallidus consistent with that observed in human HD. However, we also observed loss of dopaminergic neurons in the SN pars compacta, which is less typical as the SN pars reticulata region is more affected [36]. DeFiglia and colleagues also observed many shrunken and degenerating HTT-labeled neurons in cortical layers 5 and 6 at 2 weeks in mice that received an intra-striatal injection of an AAV1/8 vector expressing a truncated mHTT transcript (400 a.a. with 100Q) [33].
Together these results suggest that viral vector-based modeling can reproduce many of the features of the human disease but artifacts in the disease model might also be introduced by depending on the viral vector type used and thus unexpected results may need to be interpreted cautiously.
More recently, lentiviral vectors optimized for astrocytic targeting by pseudotyping with the Mokola viral envelope and using the astrocyte-specific glial fibrillary protein (GFAP) promoter to drive transgene expression has been used to investigate the effect of expression of mHTT in astrocytes [37]. Reactive gliosis and decreased expression of glutamate transporters leading to altered glutamate uptake and neuronal dysfunction were found, consistent with that found in brain samples from HD disease subjects. This provides an alternative model for examining the contribution of mHTT-mediated astrocyte dysfunction to HD pathogenesis.
1.2.2 Mutant HTT Transcripts and Expression Levels
Various N-terminal truncated mHTT transcripts have been expressed using the viral vectors as described above, with comparisons conducted relative to HTT fragments matched for size and linked to normal CAG repeat lengths (<30Q). Animals that receive control HTT vectors typically show diffuse neuronal HTT immunostaining in the striatum, and no evidence of intracellular inclusion formation or neuronal toxicity throughout the study.
Senut et al. [30], were the first to demonstrate that cumulative expression of expanded polyQ repeats throughout the life is not required to induce cell death but rather acute overexpression of polyQ is toxic to neurons in vivo. AAV2-mediated expression of expanded polyQ transcripts fused to green fluorescent protein was sufficient to induce the formation of intranuclear aggregates or large inclusion bodies as early as 5 days, with the majority of GFP-expressing neurons showing ubiquitinated aggregates by 12 days, leading to the degeneration of striatal neurons.
Several groups extended this work to characterizing models based on expression of N-terminal truncated mHTT transcripts; de Almeida and colleagues conducted a comprehensive evaluation of the relationship between mHTT expression levels, polyglutamine repeat size (19, 44, 66, or 82 CAG) and protein length (N-terminal fragments consisting of the first 171, 853, or 1520 amino acids of human HTT) [31]. Similarly, studies by deFiglia et al., and Franich et al., use constructs that fall with these ranges (the first 400 amino acids of HTT and 18 or 100Q) [33], (exon 1 of HTT and 20 or 70Q) [32]. In these studies, HD neuropathology at specific time-points ranging from 1 to 12 weeks was examined.
Nuclear HTT aggregates appeared as early as 1 week after viral vector injection, are ubiquitinated by 2 weeks [31] and progressively accumulate over the first 4 weeks. The size of the aggregates are dependent on mHTT expression levels, with lower levels associated with intranuclear and neuritic aggregates and dystrophic neurites, while larger intranuclear inclusions are found with higher transgene expression [33, 31]. Evidence of neuronal loss can occur as early as 2 weeks in the immediate vicinity of the injection site, coinciding with the peak of nuclear inclusion accumulation. A loss of anti-HTT aggregate immunostaining then coincides with extensive neuronal degeneration and reactive gliosis from 5 to 12 weeks after injection and motor impairment [32]. The time course of neuropathology is influenced by the specific transcripts expressed, with shorter mHTT fragments, longer CAG repeats, and higher expression levels resulting in an earlier onset and more severe pathology [31].
Two of these models have been used successfully to demonstrate the efficacy of RNA interference -based therapies for HD. Therapeutic silencing of mHTT with siRNA or shRNA promoted neuronal survival as well as reduced the numbers of neuropil aggregates and size of inclusions [33, 32], and prevented impairments in motor function as assessed by spontaneous forepaw usage [32].
1.3 Nonhuman Primate Models of HD Using Viral Vector -Mediated Approaches
Viral-vector mediated modeling approaches have been extended to applications to nonhuman primates. In a landmark study, Palfi et al. [38] injected lentiviral vectors expressing mHTT171-19Q or mHTT171-82Q into the dorsolateral putamen of macaque monkeys. Four injection sites were chosen covering the dorsolateral aspect of the commissural and post-commissural putamen, a region known to be involved in dyskinesia in primates with unilateral excitotoxic lesions [39]. Similar to the pathology observed in rats, mHTT171-82Q expression was associated with the formation of neuritic and nuclear ubiquitinated aggregates, loss of staining for the neuronal marker NeuN and astrogliosis at 9 weeks post-vector infusion. By 30 weeks, the size of EM48 HTT inclusions were smaller and atrophy of the putamen was clearly evident. When the vector was infused unilaterally, no changes in spontaneous behavior were observed over the 9 week period but choreiform movements were induced by apomorphine injection. Bilateral infusions of vector led to display of a spectrum of movement deficits beginning at 16 weeks and continued for up to 30 weeks including hand, leg and head dyskinesia, leg dystonia, and even tics. Furthermore, behavioral analysis of these animals showed that their neurological deficits progressed in a manner similar to the progression observed in HD patients.
The first transgenic monkey model of HD has recently been generated by Chan and colleagues [40] using a combination of transgenesis approaches and viral vector technology. Lentiviral vector s expressing exon 1 of the human HTT gene with 84 CAG repeats under the control of the human polyubiquitin-C promoter were injected into the perivitelline space of rhesus monkey oocytes. Five live newborns were delivered at full-term, with all transgenic monkeys carrying the transgenic mHTT genes but with variable repeat lengths ranging from 29 to 88 CAG. Three newborns carrying between two and four copies of mHTT developed severe symptoms, with two monkeys died shortly after birth, while the other survived for 1 month. Of the remaining monkeys, each of which caries a single copy of the mutated gene, one has only 29 CAG repeats and so shows no disease symptoms while the other has 83 CAG repeats and has developed low level deficits in movement coordination and involuntary movements such as chorea and dystonia which began 1 week after birth. HTT aggregates or inclusions were found in the striatum and cortex, with increased intensity of EM48 immunostaining correlating with high levels of mHTT with longer repeats. No obvious signs of neurodegeneration were found in striatum of the monkey that died shortly after birth.
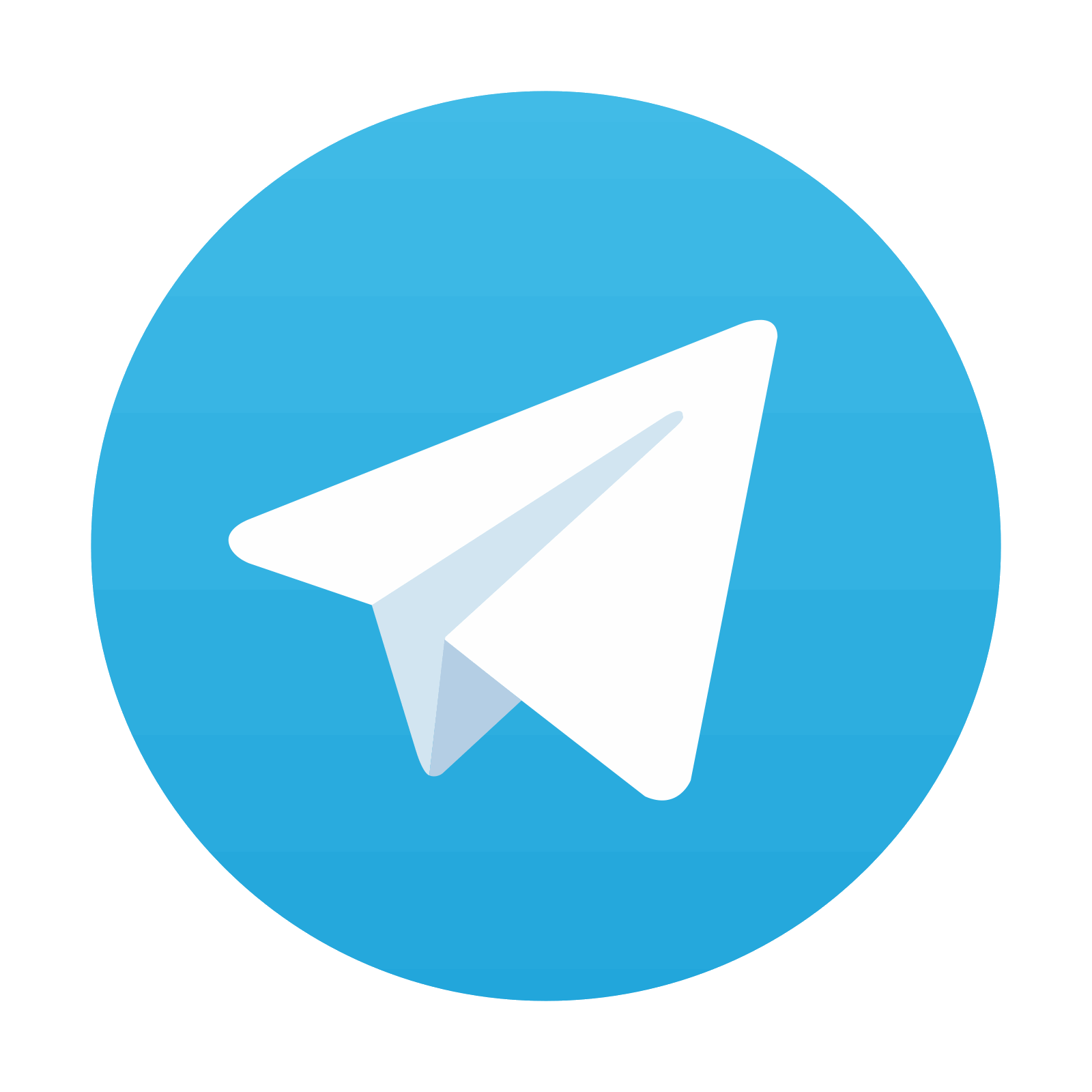
Stay updated, free articles. Join our Telegram channel
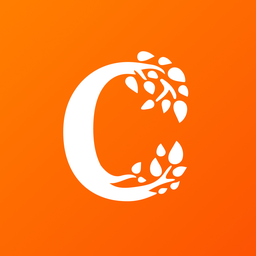
Full access? Get Clinical Tree
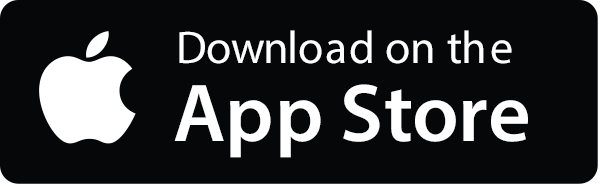
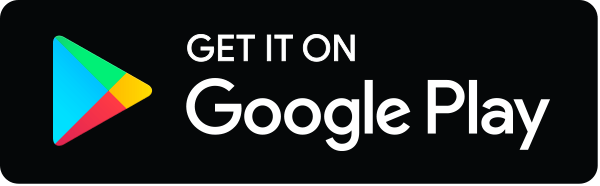