Gene Therapy for Pediatric Brain Tumors
In recent years, important progress has been made in understanding the molecular bases of disease. These tools are now being applied to the study of pediatric brain tumors and to help develop new therapies. Brain tumors result from either the overexpression or the underexpression of one or more proteins (e.g., oncogenes or tumor suppressor genes, respectively). In theory, therefore, treatment could be based on elimination of expression or function of oncogenes, or enhancement of expression or function of tumor suppressor genes in tumor cells. Gene therapy is an experimental treatment that involves introducing genetic material (DNA or RNA) into cells, and it has made significant advances over the past decade. The most common approaches for gene delivery are based on viral vectors, which have been proven reasonably safe in the central nervous system (CNS) despite occasional cases of significant morbidity in nonCNS trials. This chapter reviews the basic principles and applications of gene therapy in brain tumors. Because little work has been done on the application of gene therapy to pediatric brain tumors, much of the information presented here derives from adult trials.
What Is Gene Therapy?
Two fundamental considerations in gene therapy are (1) what gene should be delivered, and (2) how should it be delivered. In its simplest form, gene therapy is the process by which nucleic acids are transferred into cells to generate a therapeutic effect. Either replacing defective or missing genes or introducing new functions to the host′s cells can achieve this. For this purpose, the genetic material is coupled with additional regulatory sequences (promoters, enhancers, and regulatory elements) and packaged inside a gene delivery vehicle to enable transfer and expression of the intended gene product inside the cell ( Fig. 15.1 ).
How Does Gene Therapy Work?
Although conceptually straightforward, the efficient expression of foreign genes is the most crucial aspect for the success of gene therapy. The first step involves the delivery of a therapeutic gene into a cell. The simplest method is the direct introduction of DNA into target cells by physical (i.e., electroporation) or chemical (i.e., lipofection) techniques.1 This approach remains limited in its use because it is rather inefficient, and requires large amounts of DNA. Among several barriers to successful gene delivery, foreign genes or the vectors used to deliver them can trigger a range of immune responses. However, these immune responses can also be used as a gene therapy vaccine (i.e., DNA vaccines).2
The next stage for the foreign genetic material is that, once within the cell, it must escape intracellular degradation to enter the nucleus to be expressed ( Fig. 15.1 ).3,4 Therefore, gene delivery systems (vectors) were designed to protect the genetic material. An ideal vector meets three criteria: (1) it protects the transgene against degradation by nucleases in the extracellular matrix, (2) it brings the transgene across the plasma membrane and into the nucleus of the target cell, and (3) it has no detrimental effects. Currently, such vectors of gene transfer can be classified into two categories: viral and nonviral.5,6
Nonviral Vectors
Several different methods can be used to achieve gene transfer. The simplest method of gene delivery uses a physical force, such as needle injection,7 electroporation,8,9 a gene gun,10,11 ultrasound,12 and hydrodynamic delivery,13,14 employs a physical force that permeates the cell membrane, and facilitates intracellular gene transfer. However, these methods are rather inefficient in their capacity to transfer genes. Other nonviral carriers use cationic lipids, polymers, ceramic-based nanomaterials, carbon nanotubes, metal nanorods, and silica-based nanoparticles15,16 to deliver genes. Chemical approaches use synthetic or naturally occurring compounds as carriers to deliver the genetic material into cells.17 This approach involves the creation of a lipid sphere with an aqueous core.18–20 This liposome, which carries the therapeutic gene, is capable of passing the DNA through the target cell′s membrane.21,22 Synthetic and naturally occurring polymers compose another category of DNA carriers that have been used widely for gene delivery.23 In addition, there are also lipid-polymer hybrid systems.24,25 A more recent approach focuses on introducing a 47th artificial human chromosome into target cells. This chromosome would exist along with the standard 46 chromosomes without affecting their actions or causing any mutations, and it would be a large vector carrying a substantial amount of genes. A problem with this potential method is the difficulty of delivering such a large molecule to the nucleus of a target cell.26,27
Although significant progress has been made in applications of various nonviral gene delivery systems, the majority of nonviral approaches remain much less efficient than viral vectors, especially for in vivo gene delivery.
Viral Vectors
As of now, the most effective method to transfer DNA into somatic cells uses virus-based vehicles. The vehicles used for brain tumor therapy can be divided into two classes: (1) replication-defective vehicles, which we will call the vector from here on; and (2) replication-competent, or replication-conditional, oncolytic viruses, which we will call oncolytic viruses (OVs) from here on. The vector used in the first group is derived from a virus from which all or most of the viral genes have been removed to minimize virus-mediated toxicity. In the second group selected viral genes are deleted or mutated so that viral targeting and replication can occur selectively in tumor. So far, the replication-defective vectors used in brain tumor gene therapy trials have been based on retrovirus and adenovirus. In terms of replication-competent (oncolytic) viruses, the ones used in clinical brain tumors trials have been herpes simplex virus, adenovirus, reovirus, Newcastle disease virus, and measles virus ( Table 15.1 ). Hypothetically, almost any type of virus can be employed either in a replication-defective of replication-competent fashion.

Retroviruses
Retroviruses (RVs) are enveloped viruses enclosing a single-stranded RNA molecule as the genome. Following infection, the viral RNA is reverse transcribed into double-stranded DNA, which then can be integrated into the host cells genome and expressed as proteins.28,29 RVs have been primarily used as vectors, although there are preclinical studies and a likely future attempt for a clinical trial using a RV-based OV.30
The major advantages of RV vectors are their relative ease of manipulation and that they have been used widely.31 The available long-term experience with this vector has proved its low toxicity to normal brain tissue and therefore makes it a safe candidate for CNS gene therapy.32,33
A potential problem with RV vectors is that the viral genome can be inserted randomly in the host genome. If the insertion happens to be in the middle of one of the host genes, this gene will be disrupted (insertional mutagenesis). If the disrupted gene is involved in cell cycle regulation, uncontrolled cell division may occur.34,35 Other disadvantages of RV vectors are low titers, instability of the viral particles, and low transgene capacity (maximum of 7.5 kilobase [kb] foreign RNA). Another drawback of RV vectors is the requirement that the target cell must be dividing for integration and expression of viral genes. This restricts gene therapy to proliferating cells only.36,37 Although RV vectors were extensively used in early gene therapy trials, their use in more recent trials has been significantly reduced.
Adenoviral Vectors
Adenoviruses (AVs) are non-enveloped viruses with a linear double-stranded DNA genome triggering upper respiratory and eye infections in humans. Increasingly, AV vectors have become a common tool in the kit of the laboratory whenever a gene needs to be expressed in a mammalian cell, and it has also become the mainstay of gene therapy. The commonly used AV gene therapy vectors are manipulated to produce replication-deficient vectors with an insertion capacity of 10 kb of foreign DNA.
The AV vector can be modified to target it away from its usual receptor, present only in some cells,38 to other receptors present in a desired target cell.39 Once bound to its receptor, the virus enters the cell in endosomal vesicles that fuse to lysosomes.40 Next, the virus releases itself from the lysosomes and migrates to the cell nucleus. In general, viral DNA persists as an extrachromosomal element without integration in to the host genome. Usually AV gene expression is transient. To receive longer lasting gene expression, the host immune response has to be minimized. This can be achieved by eliminating exposure of gene products/proteins to non-CNS areas,41 and using AV vectors completely devoid of all viral genes (gutless vectors).42 In terms of gene delivery to brain tumors, AV vectors have been used to deliver almost any type of anticancer gene. Glioma clinical trials using AV vector-mediated delivery of the genes for herpes simplex virus thymidine kinase (HSVtk)43,44 and human interferon-β have been published so far.

In summary, AVs have been used widely as vectors in gene therapy trials, and their advantages are their relative ease of manipulation and that they can be produced at high titers. Furthermore, AV vectors are very efficient at transducing a wide variety of cells, dividing and nondividing, in vitro and in vivo. They do not usually integrate their genetic material into the host genome; rather, they replicate as episomal elements in the nucleus of the host cell with a transient gene expression, and consequently there is no risk of insertional mutagenesis.45 The limitations of AV vectors are their short-term gene expression and that they can produce toxic acute-phase responses. As clinical trials have begun to progress, it has become apparent that AV vectors may cause a significant innate immune response.46
Oncolytic Adenovirus
Adenoviruses have also been engineered to function as OVs, which replicate more efficiently in tumor versus normal cells. This selectivity can be achieved at three levels. First, every time a virus infects a cell, a robust antiviral response occurs inside the cell consisting of numerous “stress” or “danger” signals ( Fig. 15.2 ). The physiological effect of these signaling pathways is to limit the ability of the virus to replicate at high levels and thus limit the number of viral progeny that is generated and that could go on to infect neighboring cells. These “stress” signals consist of genes involved in the interferon pathways, nuclear factor κB (NF-κB), Toll-like receptor responses, protein kinase R (PKR), and others.47 Some tumor cells disabled some of these pathways since these responses tend to be pro-apoptotic and antiproliferative. Such tumor cells with disabled responses provide better targets for viral replication than do normal cells with intact antiviral responses.
Second, tumor cells have several genes involved in cell cycle regulation and apoptosis signaling disruption. These disruptions can be exploited to engineer viral mutants that cannot replicate well in normal cells with intact cell cycle control, but that will replicate in tumor cells that harbor these disruptions.
Third, viral mutants can be re-engineered so that they will target cell surface receptors present on tumor versus normal cells. So far, clinical trials of brain tumors with OVs have employed mutants that primarily use the first two mechanisms of selective tumor targeting, although retargeting of viruses—measles, for example—has been shown to be effective in the preclinical setting.48
One such oncolytic adenovirus (named ONYX-015) lacks a viral gene (E1B), which encodes for a protein that inactivates the cellular tumor suppressor protein p53. Initially, ONYX-015 was thought to target p53-deficient tumor cells, but not cells with functional p53.49,50 However, subsequent experiments with ONYX-015 revealed that the mechanism of tumor selectivity is based on a more complicated mechanism related to the nuclear export of viral messenger RNAs (mRNAs) in tumor cells.51 Other oncolytic AVs include those that target defects in the p16INK4a tumor suppressor pathway.52
Herpes Simplex Virus 1
Herpes simplex virus type 1 (HSV-1) is a human neurotropic virus, which makes it an attractive candidate for gene transfer to the nervous system. HSV-1 is an enveloped DNA virus whose genome spans 152 kb encoding for more than 80 genes. The wild-type HSV-1 is able to proceed into a lytic life cycle after infection or persist as an intranuclear episome in a latent state. Latently infected neurons function normally and are not rejected by the immune system. Although the latent virus is transcriptionally almost silent, it does possess neuron-specific promoters that are capable of functioning during latency.53 Interest in HSV-1 as an anticancer agent increased in the 1990s after initial reports demonstrated that a genetically engineered form acted as an OV in brain tumor models.54 Since then, numerous reports have detailed various types of genetically engineered mutants for glioma therapy. The two most commonly used types have revolved around deletions of the viral gene (UL39 or UL40) that encodes for the viral protein ICP6. This protein possesses the function of a ribonucleotide reductase, and recent reports have linked this defect to an ability to replicate more selectively in cells with a defect in the p16 tumor suppressor gene.55 The other type has focused on a mutation of the viral gene that encodes for the viral protein ICP34.5. This viral gene disables a host cell response that promotes apoptosis during infection.56 Mechanisms of tumor selectivity of this mutant virus have elicited several reports. In one case, the authors linked this viral defect to enhanced replication in tumor cells with elevated levels of ras activity.57 However, others have disputed this claim and have linked mutant OV replication to activity of the MEK pathway present in tumor more than in normal cells.58,59 In addition, it is also possible that tumor cells may have lost some PKR function, thus enabling this mutant virus to replicate.60
Herpes simplex virus type 1 offers several advantages for brain tumor gene therapy over other viruses: (1) it has the potential to incorporate a large load of foreign DNA; (2) it infects CNS cells with high efficiency and with low viral particle-to-cell ratio; (3) it is sensitive to antiherpetic agents like ganciclovir or valacyclovir, providing a safety mechanism by which viral replication could be eliminated; and (4) it never integrates into the host genome, which guarantees that the risk of insertional mutagenesis posed by retroviral vectors is not an issue with HSV-1 vectors. However, there are also major challenges working with HSV-1. The genetic manipulation of HSV-1 is more difficult than that with adenovirus, due to the large size of the viral genome; gene delivery could be affected due to preexisting HSV immunity of the host (60–90% of adults have been exposed to HSV-1); and the biotechnology industry′s experience with and interest in preclinical development are limited. Moreover, the potential neurotoxicity of HSV-1 vectors could cause life-threatening encephalitis from primary infection or from reactivation of latent virus.
Reovirus
This small RNA virus is endemic to the human population because most newborns get exposed to it. Usually it causes self-limiting outbreaks of respiratory and intestinal infections. A naturally occurring strain of the virus was discovered to replicate in cells with an overactive ras pathway, and this property has been exploited by attempting to define the safety and efficacy profile of this virus in clinical trials of glioblastoma multiforme (GBM) as well as other cancers.61 Like most RNA viruses, this virus can replicate to very high levels in an infected cells, and thus, in theory, it could provide an increased therapeutic “punch” since the large number of viral progeny could infect a large number of neighboring tumor cells. Some of the problems, though, that limit use of this virus relate to its small size and to its virological properties, which render genetic manipulation difficult. RNA viruses are also notoriously highly mutable, which can also be problematic for production and for use. Clinical experience is currently limited to one biotechnology venture (Oncolytics Biotech Inc., Calgary, Alberta, Canada).
Reovirus has also been used for treatment of medulloblastoma in preclinical studies. Forsyth and coworkers61 reported on the effects of treatment with reovirus in vitro and in an orthotopic model. Reovirus requires activated Ras protein for tumor cell killing. Five of seven medulloblastoma cells lines were susceptible to killing by reovirus in vitro, and, as expected, susceptibility was related to the level of activated Ras expression in the tumor cells. Similarly, these investigators demonstrated increased survival in animals injected with Daoy into the cerebellum and cerebrum and subsequently treated with a single or multiple injections of reovirus into the same site.62
Newcastle Disease Virus
The Newcastle disease virus (NDV) is an avian single-stranded RNA virus with numerous strains. Several have been adapted for growth in tumor cells, and recently a phase I clinical trial of intravenous infusion of one such mutant was reported from Israel.63 The advantages and disadvantages of this virus are similar to those of reovirus. However, differences exist: immunity to this avian virus does not exist in humans, and thus initial administration of the virus would not be limited by the host′s immune reaction. This could also be a disadvantage because the zoonotic nature of the virus and its administration into the human species could generate mutant species that become adapted to human infection and cause rapid pandemics, such as those that have occurred in the past with coronaviruses and HIV. The mechanism of selectivity of the virus for tumor cells is also not well understood. It may be due to the impaired interferon or other host cell antiviral responses in tumor versus normal cells.
Measles Virus
Measles virus, a negative-strand RNA virus, kills cells by inducing the formation of multinuclear cell aggregates known as syncytia, which result from the fusion of infected cells.64 The virus enters the cell by the binding of its H glycoprotein to the membrane-bound cell receptors signaling lymphocyte activation molecule (SLAM), found predominately on lymphocytes, or CD46, found on many cell types.65–67 CD46, or membrane cofactor protein, belongs to the family of membrane-associated complement regulatory proteins that provide protection against complement-mediated lysis and is frequently overexpressed in tumors.68 In contrast to wild-type measles virus, vaccine strains are attenuated and have a remarkable record of safety, having been administered millions of times with a significant decrease in measles incidence, morbidity, and mortality.69
Vaccine strains of measles virus have been used to kill tumor cells in several tumor types, including multiple myeloma, ovarian carcinoma, lymphoma, and, in the adult brain tumor, GBM.48,70–74 A recent report documents the efficacy of measle virus treatment in a murine model of medulloblastoma.75 Interestingly, the Edmonston vaccine strain of measles virus, unlike the wild-type virus, preferentially uses CD46 rather than SLAM as a receptor.74 This preference most likely explains the efficacy of Edmonston strains in killing tumor cells, given the high level of expression of CD46 in multiple tumor types.
Measles virus has the advantages of killing tumor cells by expression of viral genes without the need for transgene expression, and its demonstrated safety for use in humans. Disadvantages may include the acquired immunity by vaccination present in most people, although this may also limit systemic toxicity of the virus.
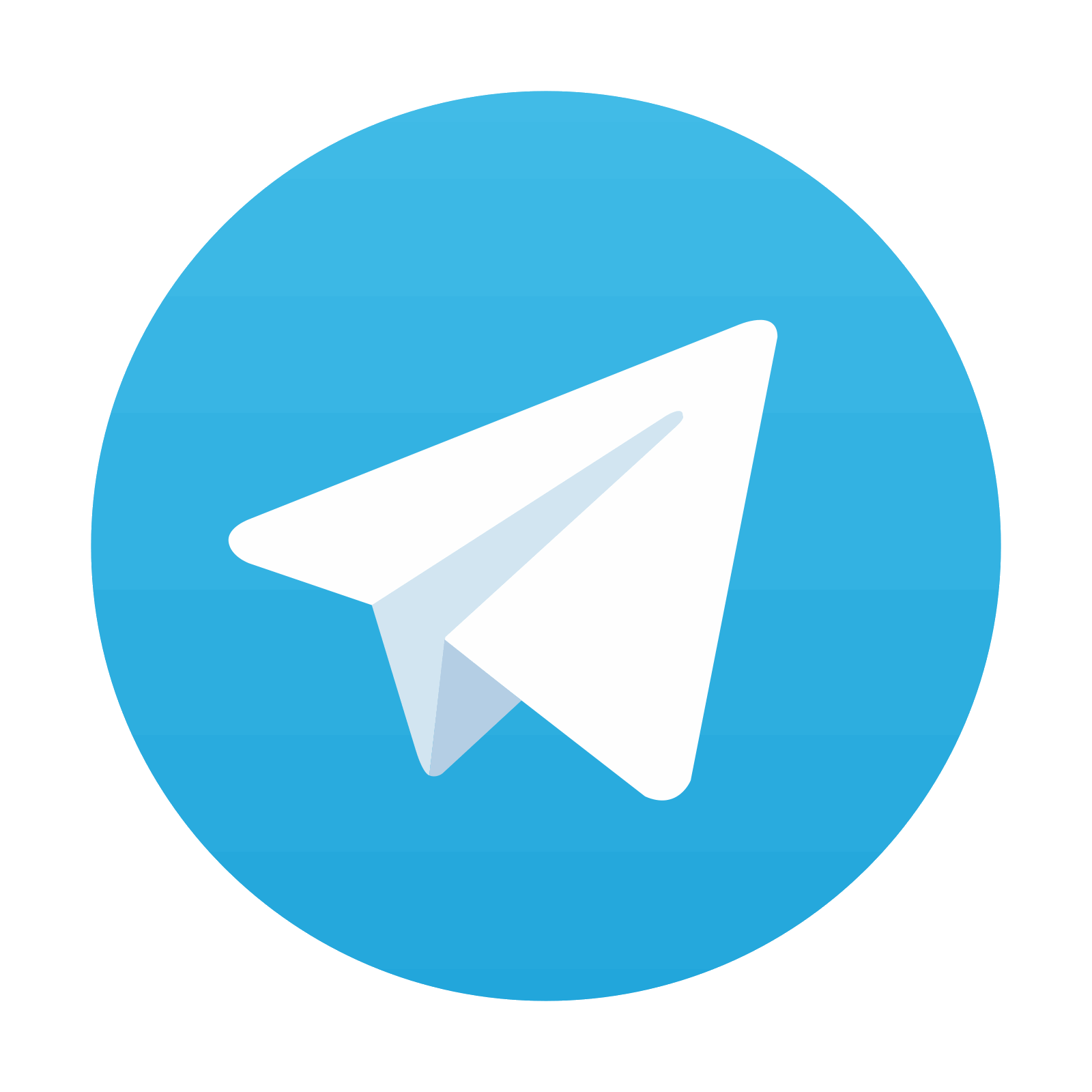
Stay updated, free articles. Join our Telegram channel
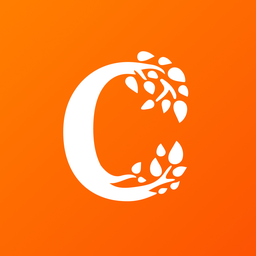
Full access? Get Clinical Tree
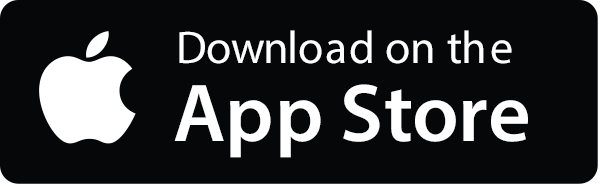
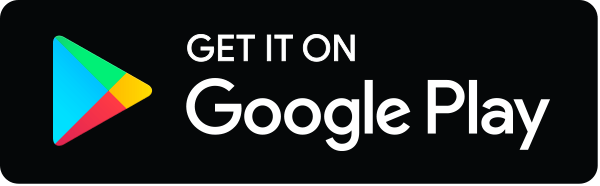
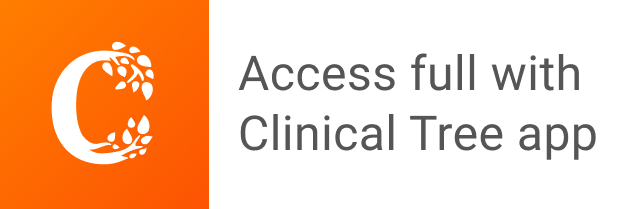