© Springer Science+Business Media New York 2016
Fredric P. Manfredsson (ed.)Gene Therapy for Neurological DisordersMethods in Molecular Biology138210.1007/978-1-4939-3271-9_3030. Gene Therapy for the Treatment of Neurological Disorders: Metabolic Disorders
(1)
University of Massachusetts Medical School, 368 Plantation Street, AS6-2049, Worcester, MA 01605, USA
Abstract
Metabolic disorders comprise a large group of heterogeneous diseases ranging from very prevalent diseases such as diabetes mellitus to rare genetic disorders like Canavan Disease. Whether either of these diseases is amendable by gene therapy depends to a large degree on the knowledge of their pathomechanism, availability of the therapeutic gene, vector selection, and availability of suitable animal models. In this book chapter, we review three metabolic disorders of the central nervous system (CNS; Canavan Disease, Niemann–Pick disease and Phenylketonuria) to give examples for primary and secondary metabolic disorders of the brain and the attempts that have been made to use adeno-associated virus (AAV) based gene therapy for treatment. Finally, we highlight commonalities and obstacles in the development of gene therapy for metabolic disorders of the CNS exemplified by those three diseases.
Key words
Gene therapy CNS Metabolic disorder s AAV Retrovirus Phenylketonuria Canavan’s diseaseNiemann–Pick disease1 Introduction
Metabolic disorder s are a vast group of diseases that can affect every cell, organ, and organism. Generally speaking, each disease that compromises the maintenance of cellular homeostasis could be considered as a metabolic disorder. For example, protein misfolding in Alzheimer disease, serotonin metabolism in major depressive disorders or nucleotide metabolism in Lesch–Nyhan Syndrome involve metabolic pathways. However, such a broad classification might be controversial and of limited use, especially considering that the metabolic contribution to the disease pathomechanism might differ substantially. Thus, we focus in this chapter on three diseases affecting classic metabolic pathways of amino acid and lipid metabolism that exemplify metabolic CNS disorders. We refer to disorders originating in the CNS as primary metabolic disorders and those originating outside of the CNS but displaying a CNS disease phenotype as secondary metabolic disorders of the CNS.
This simple differentiation between primary and secondary has substantial implications for the vector selection and the route of vector administration in particular. Primary metabolic disorders of the CNS are amendable to intracranial vector delivery with concomitant CNS transgene restriction by the blood–brain barrier (BBB). In contrast, secondary metabolic disorders of the CNS frequently require expression of therapeutic genes either restricted to a peripheral organ where the mutant gene is expressed or ubiquitously. It has also been shown, however, that muscle can serve as an ectopic biofactory of therapeutic gene products for either functioning as a metabolic sink to reduce the metabolic burden or dissemination of transgene product via the blood circulation (see Subheading 3.1).
In addition, metabolic disorders can be subdivided into mono-causative (monogenic) or multifactorial (polygenic) and inherited or acquired. For example, Canavan Disease (CD) is considered to be primary, mono-causative (monogenic) and inherited or acquired. Although patients with CD predominantly display symptoms of the CNS it has not been fully investigated if there is peripheral organ involvement as well. On the other hand, some unpublished animal studies suggest that targeting the CNS only, might be beneficial too. Nevertheless, for didactical purposes, we will consider CD as a primary metabolic disorder of the CNS in this chapter. In contrast, phenylketonuria (PKU) and Niemann–Pick disease (NPD) are secondary monogenic metabolic disorders of the CNS, with mutations in the phenylalanine hydroxylase (PAH) gene restricted to the liver and ubiquitous acidic sphingomyelinase (ASM) deficiency, respectively. Monogenic disorders serve as ideal disease models for gene therapy development, due to one gene replacement strategy and limitations in vector biology. For example, the 5 kilo base (kb) genome size of recombinant adeno-associated virus (rAAV ) often constrains space for large transgenes [1, 2].
A different genetic aspect is whether the mutation causes a gain- or loss-of-function. Loss-of-function mutations are amendable to the delivery of the native functional gene, whereas gain-of-function mutations demand reduction of the toxic gain-of-function gene product activity, which raises additional concerns of how the toxic activity can selectively be inactivated and to restore the physiologic function of the native normal gene simultaneously. This may require some dual functional vectors, meaning silencing the mutant gene but supply the normal gene function at the same time [3]. However, to our knowledge no metabolic disease of the CNS in the narrower sense with gain-of-function mutation has been targeted for proof-of-concept gene therapy experiments.
To demonstrate the challenges of gene therapy development for metabolic disorders of the CNS three enzyme deficiencies (Canavan disease, phenylkentonuria, and Niemann–Pick disease) are reviewed within this chapter that share fundamental preconditions across different disease entities, e.g., availability of transgene cDNA, animal model and vector but might demonstrate features unique to CNS metabolic disorders. One of these features is the intricacy to adjust widespread transgene delivery to peripheral organs and/or the CNS despite the BBB. For example, systemic vector administration exploits the natural ramification of the CNS blood vessels for optimal and wide spread transgene delivery but necessitates means to limit peripheral organ transduction in Canavan disease as opposed to local CNS pathologies (e.g., Huntington disease or CNS tumors) that are accessible by local vector injection. In addition, systemic vector delivery to the CNS is compromised by the first pass effect in the liver and the tight BBB, enhancing peripheral organ transduction as desired in NPD but rendering the neuron and glial cell transduction diminished. This sequestration of systemically delivered vector by peripheral organs entails the administration of higher vector doses to still efficiently transduce the CNS, which in turn may increase the risk for an immune response and the burden of vector manufacturing.
This chapter attempts to address several of these briefly introduced aspects of gene therapy development for metabolic disorders of the CNS and demonstrate how different approaches might provide improvements in these matters.
2 Lipid Metabolism Disorders
2.1 Niemann–Pick Disease (NPD)
2.1.1 Introduction
The first patient with Niemann–Pick disease (type A) was reported by the German pediatrician Albert Niemann in 1914. Niemann–Pick disease (NPD) is a rare autosomal recessive inherited disorder that is characteristic for accumulation of sphingomyelin (SPM), organomegaly and variable central nervous system (CNS ) involvement [4]. Of note, Niemann–Pick is an umbrella term that encompasses two different entities, Niemann–Pick disease type A (NPA) and type B (NPB) are classified as acid sphingomyelinase deficiencies whereas Niemann–Pick disease type C (NPC) is characterized by altered trafficking of endocytosed cholesterol [5, 6].
The onset of NPD is quite heterogeneous even within the three subclasses. For example, the occurrence of first symptoms in Niemann–Pick disease type C were reported to fall within a wide range of ages from childhood up to 60 years of age, while Niemann–Pick disease type A manifests in infancy with progressive neurodegenerative decay [7, 8]. Unfortunately, current treatment is limited to symptoms [9–11].
2.1.2 Prevalence
Demographic data for epidemiologic analysis are limited. However, the prevalence of Niemann–Pick A and B together was estimated to be 1:250,000 [12], whereas the prevalence of NPC is about 1:120,000 for live birth in Europe [5]. Some authors report increased incidence and prevalence among descendants of Ashkenazi Jews [11].
2.1.3 Symptoms
Niemann–Pick disease type A is considered to be the neurovisceral form and presents during early ages with hepatosplenomegaly, poor feeding behavior, and loss of motor function and general neurological deterioration eventually resulting in death by the age of 3 years [13]. Low HDL and hypertriglyceridemia may be present [14].
In contrast, NPB is of milder progression with symptom onset between childhood and adulthood; hepatosplenomegaly is one of the most consistent findings. Disease progression is complicated by deteriorating pulmonary function and liver dysfunction. Other symptoms may be growth restriction and impaired eye function [15, 16]. Neurological symptoms are uncommon in this subclass. As in NPA, low HDL and hypertriglyceridemia is often present [14].
Niemann–Pick disease C is both of different genetic origin versus NPD type A and B and might present quite differently. NPC can become symptomatic between infancy and adulthood [17], with cognitive dysfunction, ataxia, dysarthria and loss of language [18]. Although severity of symptoms might differ along with the onset of symptoms, most patients die between the ages of 10 and 25. Of note, even fetal NPC has been described [17].
2.1.4 Genetics
Individuals with subclasses A and B of Niemann–Pick disease share the trait that they are deficient in the enzyme acid sphingomyelinase (ASM). However, they display different phenotypes, which might be explained with no residual sphingomyelinase activity in NPD type A but low remaining enzyme activity in NPD type B [19, 20]. The gene (SMPD1) coding for ASM is localized on human chromosome 11p 15.1–15.4. Its cDNA is about 1895 base pairs (bps) long and comprises six exons, which are transcribed to 629 amino acids [7]. Several transcript variants have been identified.
In contrast, NPD type C is caused by mutations in the NPC gene that was discovered by Carstea et al. in 1997 [21]. Of those identified, 95 % of patients carry a mutation in the NPC-1 and only in about 5 % in the NPC-2 gene [22]. NPC-1 is located on human chromosome 18q11 and NPC-2 on chromosome 14q24.3. Interestingly, NPC-1 (cDNA 3836 bases) is a membrane glycoprotein predominantly located in late endosomes as opposed to NPC-2 (cDNA 455 bases), which is a soluble lysosomal protein [22]. Current hypotheses assume that both genes are involved in the same pathway that processes endocytosed cholesterol [22].
2.1.5 Pathomechanism
Acid sphingomyelinase (ASM) is predominantly found in lysosomes, where it metabolizes the membrane compound sphingomyelin to ceramide (SPM) [23, 24]. ASM follows the trafficking via mannose and mannose-6 phosphate receptors from the rough endoplasmatic reticulum (rRER) to Golgi apparatus and its final destination the lysosomes. Of note, secreted ASM can also be taken up by neighboring cells (cross-correction), which implies that partial cell transduction might be therapeutic [25]; this concept also applies to enzyme replacement therapy (ERT).
The significance of SPM metabolism to ceramide depends on the location of this catabolic step. Different stressors can translocate the hydrolysis of SPM to the cell membrane. Under these conditions, ceramide can be involved in important cell regulatory mechanisms such as autophagy, apoptosis, differentiation and cell growth [26–28]. Thus, NPD type A and B could be considered as an ASM deficiency and also ceramide metabolism disorder. A detailed review of possible molecular pathways involved in the NPD pathomechanism can be found in Smith 2008 and Schuchman 2010 [29, 30].
2.1.6 Animal Models
The first animal model s for Niemann–Pick disease type A and B were described in 1980 and 1982 [31, 32]. Although these mice presented with symptoms associated with Niemann–Pick disease, biochemical studies differed from what was found in NPD type A and B patients [33, 34]. This difference between animal and human disease manifestation can frequently be observed, questioning the authenticity of the disease imitation. In fact, Horinouchi et al. demonstrated that these mouse models did not carry any mutation in the ASM gene [35], making these mice inappropriate models for NPA and NPB studies.
2.1.7 NPD Type A Mouse Model
In 1995, Horinochi et al. engineered an acid sphingomyelinase knockout (ASMKO) mouse model by direct gene targeting [36]. This mouse is still the most commonly used model for gene therapy studies. It carries a PGK-neo expression cassette disrupting exon 2 of the ASM gene. Clinically, the ASMKO mouse displays the severe phenotype reflecting NPA. First symptoms can be noticed at 8 weeks of age with ataxia and mild tremor progressing to lethargy, non-responsiveness to stimuli and poor feeding with weight loss at 12–16 weeks of age. Death occurs between 6 and 8 months [36]. Interestingly, mice homozygous for the mutation can still breed, which facilitates the reduction of animal costs and time intensive procedures, e.g., genotyping. Molecular analyses revealed that homozygous mutant mice are deprived of residual ASM activity and accumulate sphingomyelin intracellularly. As with NPD patients, the blood lipid profile might show hypercholesterolemia but with elevated HDL. Macroscopic examination of tissue is significant for decreased size of brain and most peripheral organs; no hepatosplenomegaly was found. The CNS findings were significant for Purkinje cell loss along with cerebellar and midbrain atrophy. In addition, lipid-laden foam cells along with multilamellar inclusions are present in many organs.
Another knockout mouse model designed by Otterbach et al. displays a similar phenotype with first onset of symptoms (tremor and ataxia) at 8–10 weeks of age [37]. The affected mice present with heptosplenomegaly as seen in many patients but not in the ASMKO mouse from Horinochi et al. The disease progresses with severe dyspnea and death by 16 weeks of age, providing a mouse model with more severe phenotype. The pathology revealed an almost complete loss of Purkinje cells and accumulation of sphingomyelin and foam cells. Molecular analysis shows no transcript or enzyme activity. Interestingly, despite the more severe phenotype, which might be beneficial for the evaluation of treatment potency, this mouse model is not commonly used in gene therapy studies.
2.1.8 NPD Type B Mouse Model
In addition, a mouse model for Niemann–Pick disease type B has been engineered that only displays the characteristic visceral phenotype [38]. In view of the reduced capability of many drugs to cross the blood–brain barrier, this animal model seems to be ideal to evaluate treatment options that do not concern the brain, e.g., enzyme replacement therapy.
2.1.9 NPD Type C Mouse Model
2.1.10 Preclinical Gene Therapy Studies
Many efforts have been made to develop potential remedies for Niemann–pick disease with gene therapy being only one of them. Other concepts that have been pursued are: liver transplantation [41, 42], amniotic membrane transplantation [43], bone marrow transplantation [44–46], and enzyme replacement therapy [47]. It would be beyond the scope of this chapter to discuss all these treatment strategies. Instead, the focus will be on the aspects of ex vivo and in vivo gene therapy.
2.1.11 First In Vitro Study
Despite the natural occurrence of Niemann–Pick disease animal model s first attempts for gene therapy were not accomplished until 1992. Suchi et al. used retroviral vectors to successfully deliver the full acidic sphingomyelin (ASM) cDNA into fibroblasts from two NPA patients in vitro [48]. The resulting 16-fold increase in ASM activity and decreased sphingomyelin content underlined the success of this first attempt to correct ASM deficiency; yet only in vitro.
2.1.12 Ex Vivo Gene Therapy
Hematopoietic Stem Cell Gene Therapy
Ex vivo gene therapy relies on explantation of patient cells, in vitro transfection with the transgene and re-implantation to the patient, which was first attempted in the ASM knockout (ASMKO) mouse by Miranda et al. Male mice-derived nucleated bone marrow cells were transfected with a retroviral construct carrying the full cDNA of the human ASM (hASM). These cells were administered intravenously into 2-day-old ASMKO neonates after entire body radiation (200 cGy). Five months later, the highest ASM activity was detected in spleen, liver and lung whereas brain , heart and kidney showed insignificant difference to untreated ASM mice. Simultaneously, sphingomyelin content of the same organs was analyzed, showing a positive correlation between ASM activity and sphingomyelin reduction [49]; similar improvements were found on tissue sections. Interestingly, although there was no significant difference in ASM activity of brain in untreated versus treated mice, pathology of the cerebellum showed substantial improvement in treated mice [49]. While, this study using ex vivo gene therapy for the treatment of NPD type A/B could not rescue the phenotype and survival to the level of wild-type mice, overall, this study demonstrated the potential of hematopoietic stem cell gene therapy (HSCGT) for the treatment of NPD type A/B. However, given the limited success to improve the ASM activity in the brain, HSCGT might be more promising for NPD type B at this point.
2.1.13 Mesenchymal Stem Cell Gene Therapy
The same group demonstrated that bone marrow-derived mesenchymal stem cell (MSC) transplantation to the CNS could significantly improve the NPA and NPB phenotype. Again, cells (MSC) were transfected using a retroviral expression system carrying the human ASM cDNA. This time, however, transduced cells were injected directly into hippocampus and cerebellum of 3-week-old ASM knockout mice. Strikingly, the treatment could extend the life expectancy, improve motor function and reduce Purkinje cell loss of the cerebellum [50].
2.1.14 Combined Hematopoietic and Mesenchymal Stem Cell Gene Therapy
Later, this group combined systemic HSCGT and intracranial MSC transplantation for the treatment of the ASM knockout mouse. Three-day-old neonates were infused with hematopoietic stem cells after 400 cGy whole body radiation and the same mice were treated intracerebrally with mesenchymal stem cells at 4 weeks of age. In both cases, cells were transduced with the retroviral construct expressing the human ASM cDNA [51]. The results of this study were especially remarkable for almost 70 % of normal ASM activity in the brain of treated mice, which was in contrast to previous studies of this group [48, 51]. Unfortunately, loss of ASM activity was noted after 24 weeks post-treatment due to the development of anti-ASM antibodies [51]. These studies of ex vivo gene therapy show promising results but also bring several issues relating to the retroviral vector, e.g., efficiency, genotoxicity and in vitro cell manipulation (see Chapters 1 and 24).
2.1.15 In Vivo Gene Therapy: Systemic Vector Administration
An alternative approach to ex vivo is in vivo gene therapy , which aims to transduce cells that remain in their natural environment. Hereby, it is not necessary to harvest and re-implant cells of a patient and radiate either certain parts or the whole body, which raise additional safety concerns in clinical trial s.
Adeno-associated virus (AAV ) has become the new favorable vector for many therapeutic in vivo gene therapies, which was also used by Barbon et al. to demonstrate that intravenously delivered rAAV8hASM under the control of a liver specific promoter could correct the NPD type B phenotype in the ASM knockout mouse and performs superior to rAAV1 by about 100-fold increased ASM expression in liver. In addition, sphingomyelin clearance to almost normal levels was found in liver, spleen and lung within 8 weeks versus 12 weeks in rAAV1hASM treated mice; similar improvements were found on tissue pathology in the rAAV8hASM treated group. One drawback of this study was a humeral immune response against the rAAV capsid as well as the ASM enzyme, when ASM was expressed ubiquitously under the control of a CMV promoter. However, consistent with previous findings, liver restricted ASM expression could circumvent anti-ASM antibody immune response significantly [52]. While this study demonstrated the potential of liver restricted transgene expression for NPB it might not achieve therapeutic efficacy in NPD with CNS disease phenotype, due to the incapability of ASM crossing the blood–brain barrier.
2.1.16 In Vivo Gene Therapy: Intracranial Vector Administration
A particular route of administration for gene therapy in CNS pathologies is intracranial injection, which encompasses either intraparenchymal or intraventricular vector delivery. This could provide a powerful alternative to the usage of tissue specific promoters in systemic delivered gene therapeutics for tissue restricted transgene expression.
This was tested by Passini et al. using intracerebral injection of rAAV2-hASM in the ASMKO mouse, specifically the hippocampus [53]. Although rAAV was only injected on one side of the brain , ASM positive cells were also found on the contralateral site. The authors reasoned that this was due to axonal transport of rAAV2-hASM, which was of particular surprise given that axons in NPD type A are severely affected. Of note, 3.6–5.4 % of ASM positive cells were found on the non-injected site. Interestingly, the improvement of the CNS pathology was observed even in areas, were no ASM positive cell were found, e.g., entorhinal cortex. However, several essential regions of the brain were not reached by the local injection, e.g., thalamus [53].
Similarly, Dodge et al. injected rAAVhASM intracerebrally to attempt the rescue of gross Purkinje cell loss and improved functional outcome in ASMKO mice for NPD type A [54]. In addition, this group compared five different rAAV serotypes (rAAV1, 2, 5, 7 and 8) by unilateral injection into the deep cerebellar nucleus (DCN), with rAAV1 demonstrating the widest tissue distribution and serotype 8 the highest hASM expression. In contrast, the previously used rAAV2 serotype showed the most restricted tissue distribution and lowest protein expression, even when injected bilaterally [53, 54]. Despite the difficulties to achieve wide transgene distribution, some regions negative for hASM expression still showed decreased cholesterol accumulation. Comparing unilaterally versus bilaterally injected mice revealed improvement of motor function in both groups but bilaterally injected mice took the lead. Overall, this study revealed that on average rAAV1 was the most efficient serotype among five tested rAAVs after local injection in the ASMKO mouse CNS in terms of tissue distribution, transgene expression, and cell transduction [54].
Importantly, both studies report that intracerebral delivered rAAVhASM promotes clearing of lipid accumulation but global CNS transduction was still limited. Furthermore, intracerebral injections into the hippocampus or DCN are not efficient to recover the pathology of peripheral organs [55].
2.1.17 In Vivo Gene Therapy: Combined Intravenous and Intracerebral Vector Administration
To overcome the limitations of intracranial transgene delivery for NPD, one could inject rAAVhASM intravenously and intracerebrally at the same time. Indeed, Passini et al. showed that combined injection of rAAV8hASM intravenously and rAAV2hASM intracerebrally (2 weeks apart) benefits the overall performance of ASMKO mice over intravenous or intracerebral injection alone. Double injected animals did not only perform better in survival, motor and cognitive function in comparison to intravenous or intracerebral injected mice, the microscopic pathology was significantly improved as well [55]. Interestingly, it was found that the brain hASM level in double-injected mice was higher than in intracerebral injected animals alone despite the use of the same dose for the intracerebral injections. It has to be pointed out, that at this time, it had not been demonstrated that rAAV is capable of crossing the blood–brain barrier (BBB). As expected, intravenously injected mice showed lower levels of hASM in brain versus mice treated intracerebrally. Of note, significantly increased levels of anti-hASM antibodies were found in brain homogenates of intracerebrally treated mice but no difference from the control group in the combined injected mice, suggesting the occurrence of immune tolerance against the transgene contributed to the higher intracerebral hASM levels in the combination group [55].
In summary, the rAAV mediated hASM delivery to peripheral organs and/or the CNS has been demonstrated to improve the phenotype in the Niemann–Pick disease type A/B mouse model (ASMKO mouse). However, in order to improve safety and feasibility of this gene therapeutic approach, the transition to a large animal model would be desirable. This is especially true in view of the difference in body size between mouse and a large animal model, which is crucial for vector production, transgene delivery, and treatment efficacy.
2.1.18 Large Animal Model Gene Therapy for Niemann–Pick Disease
Salegio et al. evaluated magnet resonance imaging (MRI ) guided rAAV2hASM delivery by simultaneous unilateral brainstem and bilateral thalamus infusion in nonhuman primates (NHP) [56]. It was found that 72 % of neurons in the brainstem and 68 % in the thalamus were successfully transduced and vector distribution correlated with infusion volume. In addition, neurons distant from the injection site were transduced as well, indicating axonal transport of the vector, transgene, or transgene product. Although transduced neurons were found in remote anatomical regions, it remains to be elucidated to what extent the low transduction rate of these distant cells will contribute to clinical improvements [56].
Another study attempted the translation of the minimal effective dose of intracranial delivered rAAV1hASM, determined in mouse, to nonhuman primates (NHP) [57]. It is worthwhile noticing that this group around Bu et al. used multiple injections into cortex, subcortical structures, and cerebellum. As hypothesized, the dose correlated with sphingomyelin clearance in the ASMKO mouse. Even mice injected with the lowest dose (4 × 109 genome copies per mouse) tested, still benefited from the treatment and survived significantly longer than untreated control mice. The study group with the highest dose (1.2 × 1011 genome copies per mouse) performed best [57]. Of importance, treatment of the CNS only could significantly improve the survival, but ASMKO mice eventually died due to the visceral manifestation of the disease. This implicates that treating peripheral organs or the CNS only is not efficient for curing NPD type A and has to be taken into account for subsequent studies [55]. To translate these findings, healthy Cynomolgus monkeys were injected a total of six times into each hemisphere: motor and occipital cortex, striatum, thalamus, hippocampus, and cerebellum (total vector dose 2.6 × 1012 genome copies per brain ). Five weeks post-injection, almost all CNS regions injected showed increased hASM expression levels that were above that of the mouse group injected with the highest dose. Interestingly, the cortex of the cerebellum did not express significantly increased hASM [57]. Overall, this study demonstrates that rAAV mediated hASM delivery to the NHP CNS can provide hASM levels similar to levels found to be sufficient to treat Niemann–Pick disease in the ASMKO mouse.
2.1.19 Immune Response in Large Animal Model Gene Therapy
Among the most formidable complications in gene therapy are transgene toxicity and severe immune response. This is in accordance with several studies that failed to predict and safely translate findings in mouse or rat to large animal model s due to immune response or transgene toxicity, which emphasizes the importance of large animal studies before gene therapeutics can be applied to patients.
These aspects have been recently evaluated by Salegio et al., who conducted a safety study using rAAV2 to express hASM in naïve rats and NHPs [58].
Intra-thalamic infused rats showed increased hASM expression and activity in thalamus, cortex, prefrontal cortex, cerebellum/brainstem, and spinal cord. However, hASM activity was only significantly increased in the thalamus. In addition, monkeys were simultaneously infused into both thalami and into the brainstem and evaluated for either 3 or 9 months. Differences in hASM expression were found at 3 and 9 months post-treatment and between the low and high dose groups. Most importantly, rats tolerated the treatment without side effects, which stands in stark contrast to the rAAVhASM infused NHPs. Independent of the dose, severe side effects of neurological deterioration, weight loss, abnormal blood results were noticed. Most severely affected animals were of the high dose group that resulted in early necropsy. Tissue sections revealed cell infiltrates and gliosis along with activated macrophages, microglia, T cells and antigen-presenting cells. Furthermore, the authors could show a correlation between hASM expression and CCL5 upregulation, which might have been one of the key players in this immune response [58].
2.1.20 Challenges and Future Directions
While rAAV is considered to be nonpathogenic, there is increasing awareness in the field of gene therapy , that immunogenicity against the rAAV capsid , the transgene, or the transgene product might be the main challenge in the transition from bench to bedside. The severe side effects in NHPs reported by Salegio et al. could also have been due to transgene toxicity, which might be an artifact of using the human transgene in preclinical animal model s. However, further studies are urgently needed to develop strategies allowing for safe gene therapy in humans.
Furthermore, many metabolic diseases affect multiple cell types and organs demanding wide tissue distribution and tropism for treatment success. This also accounts for Niemann–Pick disease type A and C, which manifest in several peripheral organs and the CNS that might benefit from systemic delivery of a blood–brain barrier (BBB) crossing vector. However, serotypes tested in all studies discussed above, are known to have limitations in this regard. For example, rAAV serotype 8 displays outstanding properties in transducing hepatocytes and thus is commonly selected for liver targeting. Other serotypes (AAV1, 2, 5, and 7) have been shown to be weak performers in the transduction of cells of the CNS when administered systemically. Thus, future studies are warranted to evaluate new serotypes with wide tissue tropism, the capability of crossing the BBB and their therapeutic benefit and safety profile, in addition to approaches to circumvent severe immune responses.
3 Amino Acid Metabolism Disorder
3.1 Phenyl-ketonuria (PKU)
3.1.1 Introduction
Phenylketonuria (PKU) is a rare monogenetic disease that is caused by different mutations in the phenylalanine hydroxylase (PAH) gene [59] and was first described by Følling in 1934 [60]. The prevalence for PKU differs between countries; in the USA about 1 in 15,000 newborn have PKU [61], in Europe 1 in 10,000 [62] and in Turkey, due to the high rate of consanguinity, 1 in 4000 [63].
Clinically, untreated PKU manifests with severe mental retardation, developmental impairment, seizures, eczema, reduced hair, skin and iris pigmentation, and psychosocial problems [64, 65]. However, this is rarely seen in developed countries due to extensive screening programs of the newborn and early nutritional intervention that can reduce or even prevent cognitive impairment [65]. This also means that the patient population has shifted to more adult individuals affected by PKU, who might face other challenges than children in terms of disease management and treatment.
3.1.2 Genetics
Phenylketonuria is an autosomal recessive disorder that correlates in severity with the residual activity of the enzyme PAH. The gene coding for this enzyme is located on chromosome 12, comprises 13 exons and is 79 kilobases (kb) long. PAH is predominantly expressed in liver tissue but has also been found in other organs, e.g., kidney, pancreas, and brain [66]. Three isozymes are known in rat and two in human liver; these are all missing in classical PKU [67]. Until now, over 531 mutations (www.pahdb.mcgill.ca) in the phenylalanine hydroxylase gene have been described. Of particular interest for gene therapy is the 1358 base pair long cDNA that was cloned first from rat liver by Robson et al. and from human by Woo et al. in 1982 and 1983 respectively [59, 68] (see Chapter 1).
3.1.3 Pathomechanism
Phenylalanine hydroxylase catabolizes the hydroxylation of phenylalanine to tyrosine [69]. This irreversible reaction depends on molecular oxygen and the cofactor tetrahydrobyopterin, which is abundant in the liver [70]. It is synthesized from guanosie triphosphate (GTP) by the enzymes GTP cyclohydrolase I (GTPCH), 6-pyruvolytetrahydrobiopterin synthase (PTPS), and sepiapterin reductase (SR) [71]. Phenylalanine itself functions as an activator of PAH by inducing conformational changes [70].
Based on the residual activity of PAH, two distinct forms of PKU have been described: Classical PKU and atypical PKU. Whereas classical PKU is characteristic for no residual enzyme function at all, the atypical PKU seems to have about 5 % remaining enzyme function [72, 73]. Although the definite pathomechanism for PKU, especially the severe CNS phenotype has not been conclusively elucidated, several hypotheses have been postulated:
3.1.4 Amino Acids Transport Inhibition Hypothesis
First, in the state of hyperphenylalaninemia, phenylalanine impairs the transport of large neutral amino acids (LNAA, i.e., phenylalanine, tyrosine, tryptophan, leucine, isoleucine, and valine) across the blood–brain barrier (BBB) causing reduced levels of CNS LNAAs. This hypothesis is supported by the finding that PKU animal model s with pathologically high concentration of serum phenylalanine have reduced CNS levels of several amino acids, especially LNAAs [74]. Furthermore, a study by Pietz et al. using 1H magnetic resonance spectroscopy and phenylalanine (Phe) challenge test demonstrated that patients receiving Phe only, showed increased brain Phe and disturbed brain activity measured by electroencephalogram (EEG). In contrast, patients that received LNAAs along with Phe showed no increase in brain Phe and normal brain activity [75]. Another group could show that LNAA supplementation in the PKUENU mouse model reduced brain Phe levels [76]. However, in a prospective, double blind, crossover study in PKU patients, no correlation between Phe levels in plasma and brain could be found. Yet, LNAA supplementation reduced Phe plasma levels [77].
3.1.5 Protein Synthesis Impairment Hypothesis
Second, early experiments by Hughes et al. and Binek et al. in a pharmacologically induced PKU animal model suggested that chronically increased phenylalanine levels impair the protein synthesis of the CNS [74, 78, 79]. These findings could be restored upon administration of LNAA. However, the Phe levels remained unchanged, supporting the rational that reduced LNAA rather than elevated Phe levels contribute to the pathomechanism of PKU [74]. This finding was substantiated by Groot et al. in a positron emission tomography study using 11C-tyrosine in 16 PKU patients that showed inverse association between tyrosine influx and Phe plasma levels. Importantly, 11C-Tyrosine influx was positively associated with 11C-tyrosine protein incorporation.
3.1.6 Neurotransmitter Deficiency Hypothesis
Third, hyperphenylalaninemia causes CNS neurotransmitter deficiency. Several studies reported significant decrease in aminergic neurotransmitter, especially serotonin, dopamine and catecholamine, in the CNS and CSF of PKU patients as well as PKU mouse models [80–85]. Lou et al. reported increased Phe levels in plasma and CSF and decreased plasma levels of tyrosine and tryptophan in patients upon phenylalanine-restricted diet cessation. Interestingly, homovallinic acid (HVA) and 5-hydroxyindoleacetic acid (5-HIAA) metabolites of the dopamine and serotonin synthesis pathway were markedly decreased in three out of four patients examined upon non-restricted diet. The authors concluded that the changes in HVA and 5-HIAA might be due to impaired uptake of tyrosine and tryptophan across the BBB or at the neuronal plasma membrane caused by increased Phe levels in plasma and CSF [86]. Data from a PKU mouse model support these findings that increased Phe levels correlate with decreased levels of dopamine, serotonin and catecholamines [81, 83]. Although there is no doubt about the benefit of Phe-restricted diet in PKU treatment, decreased levels of dopamine and serotonin despite early dietary treatment have also been reported [82].
Additional pathomechanisms have been postulated. For example, oxidative stress has gained popularity in the last decade as contributing factor to many disease phenotypes (see Subheading 3.2). Kienzle Hagen et al. could demonstrate that increased levels of Phe are associated with reduced glutathione peroxidase and that Phe inhibits catalase activity [87]. A different group suggested the contribution of NADPH oxidase to the PKU disease phenotype [88]. Despite the progress that has been made to explain different factors leading to the PKU phenotype, further research has to be done.
3.1.7 Animal Models
The importance of adequate animal model s for the development of gene therapy cannot be overemphasized since it is one of the three mainstays of gene therapy development.
3.1.8 Transiently Induced Animal Models
The first animal model s were developed by feeding PAH inhibitors, e.g., p-chlorophenylalanine along with phenylalanine itself [89, 90]. This could mimic the PKU phenotype and appeared crucial to decipher the PKU pathomechanism, but was inappropriate for the development of gene therapy based therapeutics because of the missing genetic defect along with potential side effects of the PAH inhibitor [89, 91]. Even if the PAH inhibition could be maintained, cells could still express the PAH gene and provide the functioning enzyme. Any enzyme expressed from an externally introduced expression cassette would have also been inhibited, therefore making the evaluation of this gene therapeutic treatment ineffective.
3.1.9 The PAHenu Mouse
Later, Bode et al. reported an N-ethyl-N-nitrosourea (ENU) induced mutant mouse displaying symptoms of hyperphenylalaninemia. However, it was shown later that this was caused by a mutation in the GTP-cyclohydrolase gene [92, 93]. It was not until 1990, when McDonald et al. reported the creation of a mouse model (PAHenu1) carrying a mutation in the PAH gene [94, 95]. However, this mouse model failed to show the severe biological characteristics seen in human PKU patients [94, 96]. Interestingly, it was found later that the PAHhph-5 (PAHenu1) mouse carries a C to T transition at position 364 in exon 3 [97], which results in a missense mutation with the exchange of a hydrophobic amino acid by another hydrophobic amino acid (valine to alanine), which might explain the mild phenotype.
In a subsequent attempt, Shedlovsky et al. using ENU engineered two mouse strains (PAHenu2 and PAHenu3) carrying a PAH mutation on the BTBR (Black and Tan BRachyury) background. These mice demonstrated elevated Phe serum levels along with phenylketonuria. Although the PAH mRNA level as well as protein detection on WB in these two PAH mutant strains differed, they displayed the same clinical phenotype, e.g., slow growing, small head size (microcephaly), hypopigmentation and behavioral abnormalities starting at about week 2. This phenotype even deteriorated under oral Phe stress test [96]. McDonald et al. reported a C to T transition at position 835 in exon 7 of the PAH gene in the PAHenu2 mutant [97] that results in a phenylalanine to serine substitution. Interestingly, this mutation reflects the most common missense mutation of the PAH gene found in humans.
3.1.10 Preclinical Gene Therapy Studies
In the last two decades several strategies for PAH transgene delivery have been tested due to the availability of animal model s, which are still essential aspects of gene therapy development [98–106]. With the cloning of the phenylalanine hydroxylase (PAH) gene, another mainstay towards gene therapy for PKU was established [59, 68, 107–109].
3.1.11 In Vitro Studies
The first in vitro studies for PKU were conducted in 1985 by Ledley et al., who could demonstrate the functional expression of PAH mRNA and protein after NIH3T3 cell transfection with a PAH cDNA carrying expression vector. This was an important step towards virus based gene therapy [107]. Only 1 year later, in 1986, Ledley et al. successfully transduced NIH 3T3 and hepatoma cell lines using a retrovirus deprived of self-replication. Importantly, the authors demonstrated that the PAH cDNA was expressing the PAH mRNA and functional phenylalanine hydroxylase [110]. Of note, only about 10 % of the PAH activity is sufficient to accomplish therapeutic effects [107]. However, the experiments were, until that point, merely performed in vitro. Furthermore, the retroviral vector used was carrying the bacterial neo gene, which might lead to neomycin resistance in transfected cells. This is of no concern in vitro, but a major hindrance for the application in humans.
3.1.12 Ex Vivo Gene Therapy
In ex vivo gene therapy , patient cells are collected, in vitro transduced with the therapeutic gene and subsequently re-implanted.
Lin et al. transduced T lymphocytes from children with PKU with the PAH cDNA using a retroviral vector system. An important aspect of this study was the demonstration that functional PAH can be expressed in cells different than liver (heterologous) if sufficient amounts of the cofactor tetrahydrobiopterin are supplied. T lymphocytes are permeable for phenylalanine and contain small quantities of tetrahydrobiopterin that acts as an essential cofactor for the PAH catalyzed reaction. After transfection , T lymphocytes were able to catabolize phenylalanine [106]. Although this study was merely a proof-of-concept and was not further pursued, it demonstrates potential alternatives to in vivo gene therapy .
3.1.13 In Vivo Gene Therapy: Adenovirus Mediated Transgene Delivery
One of the first in vivo studies were conducted by Fang et al. who could normalize hyperphenylalaninemia in mice within 1 week after treatment using a adenoviral vectors delivering the PAH (phenylalanine hydroxylase) transgene. Unfortunately, this effect was not persistent, most likely because of a host immune response. This study also did not provide data about phenotypic changes. However, it determined that only 10–20 % of the normal enzyme activity is sufficient to decrease the phenylalanine level to normal [103].
In 1999, another study reported the normalization of blood phenylalanine (Phe) within 24 hrs using a systemically delivered replication defective adenoviruses carrying the PAH cDNA in the PAHenu2 mice [105]. The drawback of this study was that the transgene expression only sufficiently decreased blood phenylalanine for 10 days, due to a strong host immune response against the recombinant virus . Even re-administration of adenovirus -PAH failed to decrease the phenylalanine level again. Interestingly, the simultaneous administration of an immunosuppressant could extend the sufficient phenylalanine decrease in serum to 47 days [105].
Although adenovirus mediated transgene delivery could transfect cells efficiently and decrease blood phenylalanine transiently, the major drawback was a strong host immune response. In contrast, recombinant adeno-associated virus (rAAV ) has been considered nonpathogenic with low immunogenicity and high efficiency for gene therapy .
3.1.14 In Vivo Gene Therapy: Recombinant Adeno-associated Virus
The first study using rAAV mediated PAH delivery through portal vein injection, was published in 2004 by Mochizuki et al. This group could demonstrate that liver directed rAAV5mPAH delivery improves the PKU phenotype and decreases serum phenylalanine for up to 40 weeks. These encouraging findings were only weakened by the fact that the response rate of female mice was less effective versus male [102]. These results were confirmed by similar findings in the same year by Oh et al., using rAAV serotype 2 to deliver the PAH transgene [101]. Later, it was suggested that the gender dependency might be due to unknown genetic variability in the PAHenu2 animal model [111]. Furthermore, Oh et al. identified 12 genes with altered expression profiles in the untreated PAHenu2 mouse that nearly normalized upon rAAV2PAH treatment and plasma phenylalanine reduction [112]. However, the mechanism behind the gene expression profile alteration remains elusive.
Ding et al. reported the next encouraging step towards gene therapy for PKU in 2006. This group compared portal vein and tail vein injection using a rAAV2-PKU-5/8 vector in the PAHenu2 mouse. Although long-term reduction of blood Phe levels were found in both groups, portal vein injected mice performed better than tail vein injected mice (42 and 35 weeks respectively) despite fourfold lower dose in the portal vein group [100]. Of note, this study did not report any gender-dependent response to treatment, which might be due to crossing of the PAHenu2 allele in the C57BL/6 background before the start of this study [100]. In addition, several surrogate markers for liver damage (e.g., ALT, AST, γGT), inflammation (interleukin-12 and tumor necrosis factor-α) and humoral immune response against PAH were assed and reported without significant changes [99].
Another study used a pseudotyped rAAV2/8 vector in combination with a liver specific promoter to express PAH in a dose of 5 × 1011 viral particles and achieved significant decrease of blood Phe. However, the blood Phe levels were only monitored for 17 weeks post injection (endpoint of study) and could not provide longer-term expression data. In addition, interpretation of a sex-dependent response to treatment was limited due to low animal number (n = 5) and only one treated female mouse [99].
These previous studies focused primarily on transgene expression, biodistribution, safety profile and blood phenylalanine levels. However, it is worth noting that the most severe manifestation of PKU is in the CNS . Although this is most likely secondary to high blood Phe levels, the recovery of the severe CNS phenotype is of greatest importance for patients and families.
3.1.15 rAAV Mediated CNS Phenotype Improvement
In 2007, Embury et al. demonstrated that as early as 4 weeks of age, PAHenu2 mice develop neurodegenerative changes in the nigrostriatum with decreased immunoreactivity against tyrosine hydroxylase (TH), cytoplasmic vacuolar degeneration and neuronophagia by macrophage infiltration. This pathology could be reversed upon intraportal rAAV-mPAH-WPRE treatment in 10–14 weeks old mice, supporting the hypothesis that the increased Phe serum level is responsible for the underlying pathomechanism [113].
3.1.16 Muscle as a Biofactory
While common approaches target liver as the primary organ of PAH deficiency, hypotheses supporting the metabolic sink theory suggest that ectopic PAH expression might sufficiently reduce the Phe blood level and thus ameliorating the CNS phenotype by decreasing competitive inhibition of amino acid transport across the BBB (see Subheading 3.1.3).
Based on this concept, Ding et al. targeted skeletal muscle of PAHenu2 mice expressing phenylalanine hydroxylase to reduce blood Phe. However, the hydroxylation of phenylalanine requires the cofactor tetrahydrobiopterin (BH4), which has been found in sufficiently high levels in liver but not in skeletal muscle. Consequently, PAH in muscle alone did not show therapeutic effect. Conversely, using rAAV1 expressing PAH, GTPCH (GTP cyclohyrolase I, see above) and PTPS (6-pyruvoyltetrahydrobiopterin synthase, see above) via intramuscular injection of 3.5 × 1012 vector genomes sufficiently decreased Phe for at least 35 weeks [98]. The simultaneous expression of GTPCH and PTPS was necessary to guarantee sufficient levels of BH4.
Rebuffat et al. pursued the same concept targeting muscle to increase systemic PAH expression. In addition, the authors evaluated rAAV1, 2 and 8 for PAH delivery by intramuscular injection of the hindleg. However, the primary goal was not to decrease Phe via muscle restricted PAH expression rather than using muscle injections to deliver rAAVPAH to the liver. Of note, in most animals the transduction rate was highest in liver and hindleg muscle over other organs. Strikingly, strong gender dependent transduction efficiency was observed for all rAAV serotypes used. Nevertheless, the blood Phe levels could be decreased to therapeutic levels (<360 μmol/l) in males for 40–55 weeks and females for 30–35 weeks dependent on the used rAAV serotype . To extent the therapeutic effect in female mice, previously rAAV1PAH injected females were re-dosed with rAAV8PAH. This second administration of a different serotype could decrease blood Phe for additional 12 weeks [2].
3.1.17 Single-Stranded versus Self-Complementary rAAV
Another group compared intraperitoneal (i.p.) injected single stranded (ss) versus self-complementary (sc) rAAV8 of different doses and reported faster treatment response and improved long-term reduction of blood Phe for the scAAV treated group. Again, independent of the used vector, a strong gender dependent response was observed, with female mice demonstrating a weaker treatment response versus male PAHenu2 mice [114].
3.1.18 Progress in Clinical Development of PKU Gene Therapeutics
Despite impressive progress in experimental gene therapy treating PKU, the translation into a clinical setting in humans has not been accomplished. According to “clinicaltrials.gov” no clinical trial s for gene therapy of PKU have been conducted.
The closest attempts are a few clinical trial s using enzyme replacement therapy (ERT) by injecting a phenylalanine ammonia lyase subcutaneously in order to reduce overall phenylalanine. This enzyme is not expressed in humans. However, it can metabolize phenylalanine to ammonia and cinnamic acid.
3.1.19 Challenges and Future Directions
Over the last decade, different groups could demonstrate promising results in murine PKU animal model s using adeno-associated virus [98–102]. Hereby, therapeutic effects were generated by either targeting liver or skeletal muscle, raising the question if ectopic transgene expression is therapeutically sufficient.
3.1.20 Ectopic Transgene Expression
Using PKU as a model to answer the question about ectopic transgene expression, it seems that lowering the phenylalanine burden in general, proves to be therapeutically effective. This cannot account for every disease. However, studies support the hypothesis that a metabolic disorder caused by an enzyme deficiency is treatable by lowering the enzyme specific metabolite. Thus, the missing gene can be expressed ectopically as long as it is accessible by its substrate.
In the case of PKU, where the liver is the primary organ of PAH expression and target for gene therapy , some organ specific problems need to be addressed. First, in order to reduce biodistribution of the rAAV/transgene some groups attempted direct portal vein injection of rAAVPAH. This procedure itself is invasive and carries its own risks. Using tissue specific promoters or rAAVs engineering to increase tissue tropism could reduce this risk. Second, it has to be considered, that organ and tissue specific properties might hinder the transgene delivery or persistence. Under physiologic conditions the liver regenerates with an estimated cell turnover of 200–300 days [115]. rAAV genomes are usually episomal, meaning they do not integrate in the genome of the host cell. Consequently, with the replacement of each hepatocyte, the rAAV genomes vanish, leading to the observation of transgene and transgene product loss with eventual therapeutic inefficacy. Of note, the regeneration rate of the liver increases significantly after liver injury. Whether invasive methods of delivery, e.g., intrahepatic vector administration, trigger this liver-injury related regenerative mechanism needs still to be elucidated. Studies on mice, however, demonstrated rAAV genome loss in liver over time, which might be due to increased hepatocyte turn over. Targeting skeletal muscle, an organ with predominantly none dividing cells, might be a promising alternative to accomplish therapeutic long-term transgene expression.
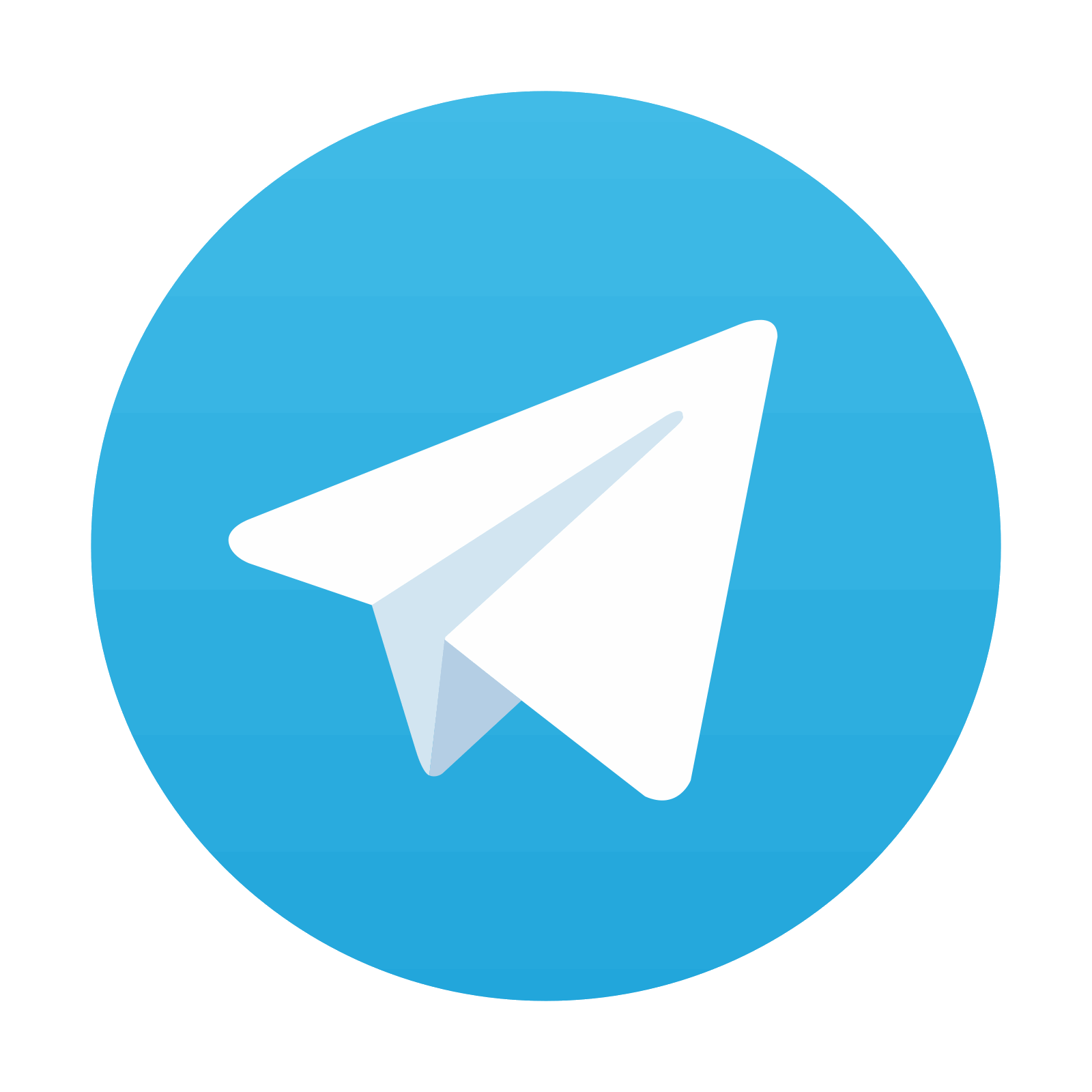
Stay updated, free articles. Join our Telegram channel
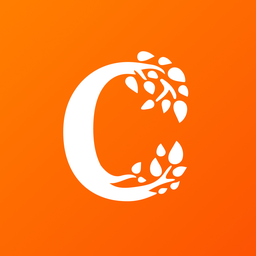
Full access? Get Clinical Tree
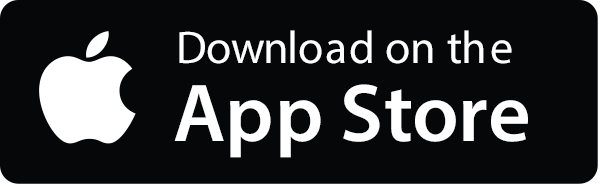
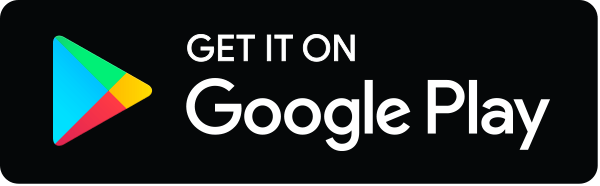
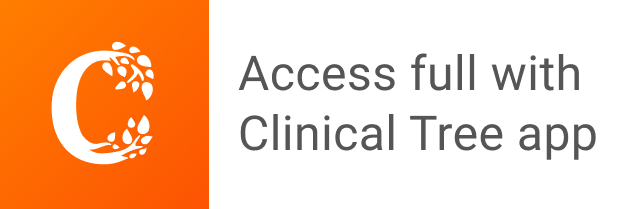