Endogenous Risk Factors
The cell of origin of glioblastoma is thought to be a neural stem cell (Box 18.1), but risk factors yielding malignant transformation of these cells remain largely elusive. Endogenous risk factors for glioblastoma other than age include a small minority of below 1% of glioblastomas that arise in patients with hereditary cancer syndromes, such as the Li-Fraumeni syndrome commonly caused by mutations in the tumor suppressor gene TP53, neurofibromatosis types I and II caused by mutations in the NF1 and NF2 genes, or the Turcot syndrome caused by mutations in the DNA repair gene APC [4, 5]. Glioblastomas in these mostly young patients are usually preceded by the diagnosis of World Health Organization (WHO) grade II or III gliomas. Families with more than one family member affected by glioblastoma are rare, thus challenging the search for susceptibility gene loci. Although a twofold risk for glioma has been reported in first-degree relatives of affected individuals [6], the familial risk association is low in consideration of the low overall glioma risk. Consequently, no high penetrance gene variants associated with glioma risk have been identified by genome-wide linkage studies among family members [7, 8]. Population-based genome-wide association studies identified five single nucleotide polymorphism (SNP) risk alleles for gliomagenesis in four genes: TP53 (odds ratio [OR] 2.70, allele frequency [AF] 0.01), EGFR (2 risk alleles: OR 1.20 and 1.25, AF 0.28 and 0.83), TERT (OR 1.35, AF 0.50), and RTEL1 (OR 1.40, AF 0.75) [9]. Of these, TERT and RTEL1 are both involved in the maintenance of telomeres and the identified risk alleles are strongly associated with older age at diagnosis and histological classification as glioblastoma [10]. These studies indicate that a distinct telomerase-associated pathomechanism is associated with the development of glioblastomas in elderly patients. The low penetrance of any risk alleles suggests that the pathophysiology of gliomas follows polygenic patterns and that these patterns follow distinct evolutionary sequences [11, 12], as discussed in more detail in Chap. 2.
Box 18.1. The Cell of Origin of Glioblastomas
The brain is the micro-anatomically by far most complex organ of the human body. Its composition of hundreds of different cell types poses the question of the cell of origin of glioblastomas. DNA is most sensitive to mutational stress during replication, but most brain cells enter a definite post-mitotic state until adulthood. A small pool of brain cells termed neural stem and progenitor cells (NSPC) retains the ability to replicate which may play a role in learning and memory as well as tissue repair after injury. Consequently, NSPC have been suggested as the most likely origin of glioblastomas [13]. In adults, NSPC have been identified in the subventricular zones lining the lateral ventricles [14], in the subcortical white matter [15] and in the hippocampi of the temporal lobes [16]. In line with the hypothesis that NSPC are the cells of origin in gliomagenesis, the majority of adult gliomas arise in supratentorial brain areas that harbor NSPC, particularly in the frontal (25.8%), temporal (19.7%), and parietal (12.2%) lobes, contrasted by lower frequencies of gliomas arising from the spinal chord (4.3%), brainstem (4.2%), cerebellum (2.9%), or occipital lobe (3.2%) [17].
Atopic disease is an endogenous factor that is associated with reduced risk for gliomas. A risk reduction by approximately 40% has been determined in a meta-analysis of 3450 patients diagnosed with glioma from eight observational studies [18]. Analyses of 911 immune function genes in germ line DNA of 1054 glioblastoma patients and 2384 controls revealed an association of glioblastoma with the IL2–RA gene encoding the regulatory T-cell (T-reg) marker CD25 [19]. The physiologic function of T-regs is to limit T-cell responses and thereby prevent autoimmunity, and lower T-reg levels are observed in patients with atopic disease. In contrast, in preclinical glioblastoma models higher frequencies of T-regs decrease survival and contribute to the immunosuppressive microenvironment that prevents immunologic antitumor responses [20, 21]. The challenges that T-reg and other immunosuppressants pose to upcoming immunotherapy approaches against gliomas are discussed in more detail in Chap. 12.
Exogenous Risk Factors
Exogenous risk factors for the development of glioblastomas are widely elusive. No association with exposure to cancerogenic agents or smoking has been reported. Risk associations of brain cancer and gliomas with ionizing irradiation have been studied, but data on the more specific association with glioblastoma have not been reported. In a cohort of 105,427 atomic bomb survivors including 56 patients with gliomas, a linear dose–risk relationship with an excess relative risk (ERR) of 0.56/Gy (95% confidence interval [CI] -0.2-2.0) has been reported for gliomas at moderate dose levels with a time lag of more than 15 years, and this relationship was less pronounced with increasing age at exposure [22]. In a population-based study in 14,361 long-term survivors of childhood primary brain tumors, high-dose irradiation (30–44.9 Gy) yielded an excess absolute risk of 19.3 per 10,000 patient years for the diagnosis of gliomas (N = 40, including 10 glioblastomas) [23]. A third population-based study investigated the long-term risk for brain tumors during a median follow-up period of 40 years after radiotherapy of the skull. A total of 10,834 patients were treated for tinea capitis during childhood with up to 6 Gy. Comparison with 5392 siblings as well as a matched population group yielded an ERR of 2.6 (95% CI 0.8–8.6) for any gliomas and the ERR for “high grade gliomas,” referring to anaplastic gliomas or glioblastomas, followed linear kinetics at 1.98/Gy (95% CI 0.73–4.69) [24, 25]. Doses of diagnostic computed tomography (CT) scans of the brain reach doses in the range of 30–40 mGy and should be considered too low to increase glioma risk, but recent epidemiology studies with a maximum follow-up in the range of 20 years suggest that the relatively higher biological activity of low-energy x-radiation from CT scans may yet yield relevant effects: In a population-based study among 176,587 children receiving diagnostic CT scans, the ERR for gliomas was 0.019/mGy [26] and a case–control study reported an increased risk for gliomas among patients with a positive family history for any cancer that received >3 repeat CT scans (OR 3.74, 95% CI 1.24–11.28) [27]. A third population-based study in 11 million people in Australia including 680,000 children and adolescents exposed to diagnostic CT scans defined an incidence rate ratio of 2.44 (95% CI 2.12–2.81) for brain cancer based on 210 patients exposed to brain CT that suffered from brain cancer subsequently and these values decreased with increasing age at first exposure [28], but the specific risk for glioblastoma was not reported.
The spread of mobile phone use at the end of the 1990s prompted extensive studies of brain tumor risk, but no definite association of mobile phone use with gliomagenesis has been reported [9, 29]. Occupational exposure to extremely low-frequency electric fields was studied over decades, including one study following 20,141 Swiss railway workers for 30 years [30] and one Dutch study following 120,852 people with high exposure to electric fields for 17 years [31], but no association with lifetime risk for glioblastoma or other brain cancers was observed. The INTEROCC study including 2054 glioma cases and 5601 controls likewise reported no association of exposure to extremely low-frequency electric fields with lifetime glioma risk, but there was an association of glioma risk with exposure 1–4 years prior to diagnosis [32].
Viral oncogenesis and particularly an oncogenetic or oncomodulatory role for cytomegalovirus (CMV) in gliomagenesis have been postulated, because some of the CMV gene products interact with glioblastoma core signaling pathways, but experimental evidence to confirm this role for CMV is scarce [33, 34].
Screening and Prevention
Biomarkers for detecting glioblastomas in a systematic screening program are not established and concerns exist about the wide use of magnetic resonance imaging (MRI), because detection of incidental imaging changes of unclear clinical significance may yield unnecessary operations [35]. Low incidence rates of glioblastomas would yield high rates of false-positive MRI lesions, whereas rapid growth renders the time window for detection in the range of a few weeks to months [36]. The recent identification of single nucleotide polymorphism (SNP) risk alleles sheds light on the perspective of a genetic blood test to estimate glioblastoma risk [36]. Such a test would be less resource-intense than MRI and could preselect high-risk patients for MRI to reduce the rate of false-positive MRI, but clinical risk factors that could add to such a genetic risk assessment are elusive, thus disabling preventive measures [36]. Detection of smaller tumors facilitates safe resection and thereby improves outcome in selected study populations [37–39], but, finally, there is a lack of evidence to support the notion that earlier detection and earlier treatment of glioblastoma improve outcome.
Diagnosis and Disease Monitoring
Clinical Presentation
Tumor location, epilepsy, and the extent of edema and tissue destruction determine the disease course. Therefore, there is no typical clinical presentation of glioblastoma. Despite the fatal prognosis of glioblastoma, quality of life and cognitive functioning may be preserved or improved by the standard treatment options discussed further below, even in the frail elderly population, but once first-line treatments have failed, decline of quality of life and cognition is usually rapid and may be severe [40–43].
In adults, approximately half of all glioblastomas infiltrate multiple lobes and multifocal growth occurs in approximately 5% [44]. Approximately 20% of all patients with glioblastoma initially present with sensorimotor symptoms, and initial growth in the speech-dominant, i.e., mostly the left hemisphere yields aphasia as the presenting symptom in approximately 5% of glioblastoma patients [45].
Transient aphasia and transient sensorimotor deficits termed Todd’s paresis may result from epilepsy, particularly from seizures that evolve from the temporal lobes [46]. Epilepsy precedes the initial diagnosis of glioblastoma in 24–68% of patients and develops in additional 19–38% later during the course of the disease [46–49]. Epilepsy at diagnosis is associated with longer survival, probably because tumors are diagnosed earlier during the disease course, as indicated by an association with smaller tumor size, and because epilepsy is associated with younger age as well as cortical location, thereby yielding good surgical resectability [45, 46]. Anticancer effects of anti-convulsant drugs have been proposed, particularly for valproic acid [50], but pooled analyses of prospectively collected data of 1896 patients from four recent clinical trials do not support this hypothesis [51].
Less than one-third of all patients with glioblastoma initially present with headaches [45], mostly as a result of increased intracranial pressure that typically presents at night or at awakening with a dull character. Other symptoms of increased intracranial pressure including dizziness, fatigue, nausea, vomiting, and neurocognitive slowing may be present at diagnosis. Almost generally, these symptoms evolve during the disease course. Symptoms of increased intracranial pressure may be ameliorated by the use of steroids, typically dexamethasone at up to 12 mg daily as single dose in the morning [52].
Diagnosis of frontal lobe glioblastomas may be delayed, because frontal tumors can mimic psychogenic disorders or may be mistaken for the physiologic aging process by presenting with mood disorders or personality changes. However, survival of patients with frontal lobe glioblastoma is longer compared to patients with glioblastomas arising from the parietal or temporal lobes [53], probably due to an association with favorable prognostic features such as younger age, mutations in the genes encoding isocitrate dehydrogenase (IDH) 1 and 2 [54, 55], and higher rates of complete resection of contrast-enhancing tumor [56].
Leptomeningeal dissemination of glioblastoma cells may cause headache and mimic spinal disease such as painful nerve root compression or myelitis. Clinical suspicion and diagnosis of leptomeningeal dissemination are rare and, if at all, usually occur late during the disease course [57], but in one autopsy study 5 of 25 patients had leptomeningeal metastases [58]. Risk factors reported from the few systematic studies on leptomeningeal dissemination in glioblastoma include incomplete or multiple resections, ventricular entry or proximity of the tumor to the ventricular system, younger age, male gender, and gains at the 1p36 chromosomal region [59–63].
Glioblastomas arising from the brain stem typically present with combinations of cranial nerve palsies, dysphagia, or occlusive hydrocephalus. Brain stem glioblastomas are rare in adults, but account for the majority of pediatric glioblastomas [64].
Glioblastomas exhibit a particular tropism to the brain and only few anecdotal cases of distant organ metastases, mostly to lung, pleura, lymph nodes, bone, and liver, have been reported [65]. Consequently, follow-up scans of the entire body are dispensable in glioblastoma patients and transplantation of organs from donors with glioblastoma is per se feasible, because the risk of cancer transmission is minimal [66, 67]. However, organ donation requires sudden intracranial events such as hemorrhage that lead to brain death, or gradual death of intubated patients to enable non-heart-beating organ donation [68], but both modes of death are uncommon in glioblastoma, because patients mostly die as a consequence of gradual decline in a home or hospice setting.
Imaging
New onset neurological symptoms are commonly followed by MRI as part of a neurological work-up. CT may be performed when MRI is not available or not possible, e.g., because of cardiac pacemakers or other metallic implants, or when acute hemorrhage is suspected, but the sensitivity of CT to detect glioblastoma-specific changes is inferior to MRI. On MRI, glioblastomas typically appear as diffuse masses that are characterized by contrast enhancement at the margin that marks disruption of the blood–brain barrier. Furthermore, hypointensity on T2-weighted images at the tumor core marks necrosis, and hyperintensity on T2-weighted and fluid-attenuated inversion recovery (FLAIR) images of the surrounding brain parenchyma is a correlate of edema or non-contrast-enhancing tumor tissue (Fig. 18.2).


Fig. 18.2
Neuroimaging features of glioblastoma. Magnetic resonance imaging (MRI), T1-weighted gadolinium-enhanced (a), fluid-attenuated inversion recovery (FLAIR, b).
Radiographic diagnostics of glioblastoma have been refined by diffusion/perfusion- and susceptibility-weighted MRI sequences, T1 contrast subtraction maps, and MR spectroscopy, and tractography or functional MRI has been implemented in many centers to improve maximal safe resection [69]. Overall, advances in imaging technology have improved the discrimination of glioblastoma from other contrast-enhancing lesions such as metastases, from non-brain tumors, primary central nervous system lymphomas, or abscess or inflammatory lesions [70–74]. Detection of the oncometabolite 2-hydroxyglutarate, which is metabolized by mutant isocitrated dehydrogenase (IDH), may enable the diagnosis of IDH-mutated glioblastoma by MR spectroscopy [75]. Positron emission tomography (PET) utilizing amino acid tracers, typically O-(2-[18F]fluoroethyl)-l-tyrosine (FET), is increasingly applied to identify hot spots of increased metabolism and presumably highest tumor grade before biopsies [76]. The developments of imaging technology are discussed in more detail in Chap. 1, but we emphasize that despite these advances, the appearance of glioblastoma on imaging scans can vary considerably, thus making tissue-based diagnosis yet indispensible [77].
Histopathology
The definite diagnosis of glioblastoma is made by histology. Glioblastomas are assigned to the highest grade of the 2007 World Health Organization (WHO) classification of primary brain tumors, i.e., grade IV, which is defined by the presence of necrosis and microvascular proliferation. Other signs of malignancy shared with anaplastic gliomas, which are assigned WHO grade III, include anaplasia, high mitotic rates, and invasiveness [78]. Furthermore, two rare histopathological glioblastoma variants, giant cell glioblastoma and gliosarcoma, have been included as distinct tumor entities in the 2007 WHO classification of primary brain tumors and account for approximately 2% of all WHO grade IV gliomas [78]. Extensive lymphocytic infiltration is present in giant cell glioblastoma, but a putative clinical significance of this feature, e.g., for the application of immunotherapy approaches, is not known. The multinucleated phenotype of giant cell glioblastoma is associated with the loss of TP53 and high expression levels of aurora B [79]. Giant cell glioblastoma is associated with slightly better prognosis than glioblastoma when adjusting for age, gender, race, tumor size, surgical extent, and radiation therapy use (HR = 0.76, 95% CI 0.59–0.97) [80]. Gliosarcomas may resemble meningiomas macroscopically and are characterized by a prominent mesenchymal metaplastic histological appearance. Extracranial metastases occur more frequently and the prognosis of gliosarcoma is probably slightly worse compared to glioblastoma (HR = 1.17, 95% CI 1.05–1.31) [81].
Recently, overlap of the genomic and epigenetic characteristics of central nervous system primitive neuroectodermal tumors (CNS-PNET) and a distinct molecular glioblastoma subtype that is characterized by point mutations in G34 of histone H3.3 have been identified in a cohort of 81 G34-mutant CNS tumors, thus suggesting that CNS-PNET and G34-mutant glioblastomas comprise a single biological entity [82]. Other histopathologic glioblastoma variants that have been suggested include small cell astrocytoma, fibrillary glioblastoma, and granular cell astrocytoma [83], but the biological significance of these histopathological diagnoses is less clear. Glioblastomas that are preceded by the histological diagnosis of WHO grade II or grade III gliomas are termed secondary glioblastoma, but the molecular marker-based classification of gliomas discussed further below and in more detail in Chap. 2 has widely substituted this terminology and will complement the next edition of the WHO classification of primary brain tumors [36].
Molecular Classification
The evolving landscape of molecular heterogeneity of glioblastoma currently defines at least 7 molecular subtypes based on the integrated analyses of genomic, epigenetic, and gene expression data [12, 54], but treatment concepts that take these molecular classifications into account are yet to be established. It is also of note that the biomarker with the strongest impact on clinical decision making, i.e., hypermethylation of the promoter of the O6-methylguanyl DNA methyltransferase (MGMT) gene, was not detected as a distinguishing feature of the molecular glioblastoma subtypes that were identified by unsupervised integrative analyses of (epi-)genome-wide analyses. MGMT is a DNA repair protein that counteracts DNA alkylation by chemotherapy and MGMT gene silencing due to promoter methylation predicts benefit from alkylating chemotherapy in glioblastoma [84–87].
Besides MGMT testing, a rough dual prognostic categorization of glioblastoma based on the presence of distinct point mutations of IDH–1 or –2 has entered clinical practice [36]. IDH–1 or –2 mutations are early events during the evolution of glioblastoma that are strongly associated with younger age and longer overall survival, and these glioblastomas commonly evolve from histopathological WHO grade II or grade III gliomas [88, 89]. IDH mutations are present in approximately 5–10% of all glioblastomas and are virtually absent in patients aged 65 years or older. The most common IDH mutation making up approximately 90% of all IDH mutations in glioblastoma is IDH1R132H, which can be detected by immunohistochemistry [90], but detection of other IDH1 or IDH2 mutations requires gene sequencing.
In 2010, four glioblastoma subtypes termed proneural, neural, classical, and mesenchymal were defined based on the differential expression of 840 genes in 200 glioblastoma samples, and IDH mutant glioblastoma was identified as a subgroup of the prognostically favorable proneural gene expression subtype [91]. Subsequent methylome-wide analyses of 210 glioblastoma samples that included 59 pediatric glioblastomas identified six glioblastoma subtypes of which only IDH mutant tumors retained the favorable prognosis of the proneural gene expression pattern [54], and these IDH mutant glioblastomas can be further subclassified based on a distinct epigenetic pattern designated glioma CpG island methylator phenotype (G-CIMP) [92, 93]. IDH mutant glioblastomas that do not exhibit the G-CIMP gene methylation pattern are instead characterized by the activation of cell cycle genes as a result of cyclin-dependent kinase 4 (CDK4) gene amplifications and cyclin-dependent kinase inhibitor 2A (CDKN2A) gene deletions, and the prognosis of these patients is unfavorable [12]. IDH wild-type glioblastomas that cluster with proneural gene expression include two glioblastoma subtypes that affect children and adolescents almost exclusively and that are characterized by G34 and K27 mutations of histone H3 (H3F3A). Mutations in IDH and H3F3A G34 or K27 are mutually exclusive, but share a strong association with mutations in the tumor suppressor gene TP53 [54]. A third IDH wild-type glioblastoma subtype with proneural gene expression and unfavorable prognosis was termed receptor tyrosine kinase (RTK) I and is associated with high expression levels of the gene encoding platelet-derived growth factor receptor alpha (PDGFRA) and with gene deletion of CDKN2A [54]. The RTKI subtype may occur at any age. Furthermore, two non-proneural subtypes with unfavorable outcome have been defined, and these cluster with (i) the classical gene expression subtype characterized by EGFR amplifications and CDKN2A deletions (designated RTKII), and (ii) the molecularly more heterogenous mesenchymal gene expression subtype [54].
Other molecular markers in glioblastoma include activating mutations in the promoter region of telomerase reverse transcriptase (TERT) and a specific deletion in the ligand-binding domain of EGFR designated EGFRvIII or delta–EGFR yielding ligand-independent receptor activity. TERT promoter mutations are associated with older age and particularly poor prognosis in IDH wild-type glioblastoma [94, 95]. The EGFRvIII deletion is present in approximately half of all RTKII (classical) subtype glioblastomas with EGFR amplifications [96] and is thought to confer poor prognosis by interacting with cells that bear amplified wild-type EGFR [97], but immunologic targeting of EGFRvIII utilizing a peptide vaccine directed against EGFRvIII failed to improve survival in the double-blinded phase III trial ACT-IV (NCT01480479). Despite such drawbacks and the fact that treatment choices are not affected by the detailed molecular characterization of individual tumors, the ongoing segregation of glioblastoma subtypes will eventually yield more personalized approaches to improve the outcome of glioblastoma patients, as discussed in more detail in Chap. 3.
Standard First-Line Therapy
Surgery
Tissue for establishing the diagnosis of glioblastoma is indispensible, and therefore, surgery is the first step in the therapeutic cascade for suspected glioblastoma. Complete microsurgical resection of contrast-enhancing tumor (CRET) is the standard of care whenever safely possible, but biopsies are performed in multifocal disease or if major disability upon tumor resection is expected due to tumor location in functionally vulnerable areas of the brain [77]. Biopsies are mostly performed stereotactically, but open biopsies may be preferred in cases where the tumor is well accessible, because a larger amount of tissue can be obtained for molecular diagnostics and because the risk of sampling errors is lower.
Whether surgery for mere reduction of tumor volume rather than aiming at complete resection improves outcome is under debate, because no prospectively collected data support that the higher risk of open surgery compared to stereotactic or open biopsies pays off in terms of overall survival. Various retrospective cohorts do, however, indicate benefit from incomplete tumor resection, including one cohort comprising 500 consecutive patients from a single institution, which identified a threshold of approximately 80% reduction of tumor volume for survival benefit from surgery [38], but such estimates are rarely applied in clinical practice for pragmatic reasons, and all these uncontrolled series have been criticized for major biasses.
In contrast, the therapeutic value of CRET is well documented and clinically applicable for both planning surgery and estimating the patients’ postoperative prognosis. In a randomized controlled phase III trial that was designed to assess the value of 5-aminolevulinc acid to improve the extent of resection in 322 patients with suspected anaplastic glioma or glioblastoma, a prespecified stratification by extent of resection demonstrated that CRET improved overall survival compared to patients with residual contrast-enhancing tumor on postoperative MRI (17.9 months [95% CI 14.3–19.4] vs. 12.9 months [95% CI 10.6–14.0]) [39]. CRET was also associated with improved outcome among the 371 elderly patients with anaplastic astrocytoma (N = 40) or glioblastoma (N = 331) that were treated in the phase III NOA-08 trial. Besides extent of resection, the prespecified survival model controlled for age, histology, MGMT promoter status, and study treatment discussed further below, i.e., postoperative radiotherapy or chemotherapy with temozolomide [85]. Means to improve the extent of resection are discussed in more detail in Chaps. 4–6.
Chemoradiotherapy
The standard postoperative therapy regimen for patients with newly diagnosed glioblastoma in good general condition is radiotherapy (30 × 2 Gy = 60 Gy of the involved field) plus daily concomitant temozolomide at 75 mg/m2, followed by an interval of approximately 4 weeks without therapy and up to 6 cycles of temozolomide at 150–200 mg/m2 on 5 out of 28 days [77]. The randomized phase III trial establishing this regimen was a transatlantic cooperative effort of the European Organisation for Research and Treatment of Cancer (EORTC) and the National Cancer Institute of Canada (NCIC) and demonstrated improvement of median overall survival from the adjunct of temozolomide to radiotherapy alone by 2.5 months (hazard ratio [HR] 0.63, 95% CI 0.52–0.75) [98], but benefit from temozolomide among patients without MGMT promoter hypermethylation was only marginal [84]. Furthermore, patients aged 66–70 years appeared not to benefit from the adjunct of temozolomide to radiotherapy in post hoc analyses (HR 0.78, 95% CI 0.50–1.25, p = 0.29) [99], and patients aged older than 70 years were not included in this trial [98]. The efficacy of combined chemoradiotherapy versus radiotherapy alone in elderly patients with newly diagnosed glioblastoma in good general condition will be defined by the recently completed NCIC CE6 phase III trial (NCT00482677), but to date combined chemoradiotherapy should probably only be considered in fit elderly patients with a methylated MGMT promoter [77]. Furthermore, two phase III trials in 811 patients with newly diagnosed glioblastoma [100] and in 223 patients with recurrent anaplastic astrocytoma or glioblastoma [101], respectively, failed to demonstrate benefit from a dose-intensified temozolomide regimen utilizing 80–100 mg/m2 on 21 out of 28 days compared to standard temozolomide dosing at 150–200 mg/m2 on 5 out of 28 days, but more toxicity was observed with the dose-intensified regimen in both trials, thus making dose-escalation strategies obsolete [77].
Therapeutic Approach in Elderly Patients
Radiotherapy
A randomized controlled trial that included 83 patients aged 70 years or older with newly diagnosed glioblastoma (N = 81) or anaplastic astrocytoma (N = 2) demonstrated that postoperative radiotherapy in elderly patients improves overall survival compared to best supportive care alone (median overall survival: 29.1 vs. 16.9 weeks, p = 0.002) without detrimental effects on quality of life or cognition [40], and a population-based retrospective analysis of almost three thousand patients with glioblastoma aged 71–98 years (median age 76.9 years) further supports these results (HR 0.43, 95% CI 0.38–0.49) [102]. In consideration that daily traveling to receive radiotherapy is too much of a burden for some of the sometimes clinically severely affected elderly patients, a randomized trial in 95 patients aged 60 years or older compared the standard radiotherapy regimen (30 × 2 Gy = 60 Gy) with hypofractionated radiotherapy (15 × 2.66 Gy = 40 Gy) and demonstrated comparable activity of both regimens (HR 0.90, 95% CI 0.60–1.35, p = 0.61) [41]. The rationale for this study was further underpinned by results from the intention to treat population of the three-armed randomized Nordic trial, which included a standard radiotherapy arm with numerically even inferior survival compared to a hypofractionated radiotherapy arm with 10 × 3.4 Gy = 34 Gy (overall survival 7.0 vs. 5.2 months, p = 0.02), probably because standard radiotherapy was discontinued prematurely by 22 of 94 patients, compared to only 2 of 119 patients who discontinued hypofractionated radiotherapy [86].
Chemotherapy
Two randomized phase III trials, the Nordic trial and the NOA-08 trial, established postoperative chemotherapy with temozolomide as an alternative to radiotherapy in elderly glioblastoma patients with hypermethylation of the MGMT promoter [85, 86]. The NOA-08 trial randomized 412 patients aged 65 years or older with primary diagnosis of glioblastoma or anaplastic astrocytoma, out of which 373 patients received at least one dose of standard radiotherapy or temozolomide at a dose-dense schedule of 100 mg/m2 given on days 1–7 every other week (1 week on/1 week off) to be included in efficacy analyses and demonstrated non-inferiority of temozolomide to standard radiotherapy after adjustment for histological diagnosis, extent of resection, age, and MGMT promoter methylation status (HR 1.09, 95% CI 0.84–1.42) [85]. The Nordic trial randomized 342 patients aged 60 years or older with primary diagnosis of glioblastoma to receive one of two radiotherapy regimens or temozolomide dosed at 150–200 mg/m2 on 5 out of 28 days. Survival analyses of the intention to treat population demonstrated similar efficacy of temozolomide (N = 93) versus hypofractionated radiotherapy (N = 98) (HR 0.82, 96% CI 0.63–1.06) after adjustment for age, type of surgery (biopsy versus resection), and WHO performance score [86]. In consideration that dose-intensified temozolomide regimens yield higher toxicity at similar efficacy in glioblastoma (see above), temozolomide at 150–200 mg/m2 on 5 of 28 days is the standard dosing regimen that should be applied in patients with newly diagnosed or recurrent glioblastoma [77].
Management at Recurrence
To date, no standard of care has been defined for recurrent glioblastoma [77] and treatment options are overall limited [103]. The choice of treatment depends not only on previously administered therapies, but is substantially influenced by tumor characteristics, availability, and local preferences. The efficacy of any established treatments for recurrent glioblastoma is modest, particularly in consideration that patients recruited to randomized controlled trials for recurrent glioblastoma comprise a preselected population with more favorable prognosis, excluding a substantial fraction of patients that are already heavily impaired at first progression. Therefore, best supportive care focusing on the amelioration of symptoms including psycho-oncological interventions may be adequate in many patients already at first progression [77].
Disease Monitoring
MRI, typically performed every 2–3 months, is the standard method for the diagnosis of recurrent disease. Unimodal response assessment based on contrast enhancement was the standard for almost two decades [104], but pseudoresponse, i.e., rapid normalization of contrast enhancement under anti-angiogenic treatment, and pseudoprogression, i.e., an increase in size of contrast-enhancing lesions after radiotherapy, challenge MRI-based response assessment in glioblastoma [105]. Therefore, multidimensional criteria defined by the Response Assessment in Neuro-Oncology (RANO) working group, which incorporate time and type of treatment, T2/FLAIR, corticosteroid use, and clinical characteristics, have been widely adopted [106] and emphasize the requirement of interdisciplinary boards for treatment decisions in glioblastoma patients (Chap. 1). More recent developments suggest that O-(2-[18F]fluoroethyl)-l-tyrosine positron emission tomography (FET-PET) may overcome some of the limitations of MRI [107–109], but FET-PET is not part of the standard work-up of glioblastoma.
Surgery
The role of repeat surgery is under debate because of a lack of randomized controlled clinical trials, availability of subgroup analyses of only few prospectively collected datasets, and high probability of selection bias for surgery among patients included in retrospective analyses. The largest available dataset was derived from a prospective registry study including 764 patients with glioblastoma diagnosed and treated 2004–2010, among which repeat surgery was performed in approximately one-third of patients. No association of repeat surgery with overall survival was noted in this cohort (HR 1.02, 95% CI 0.77–1.34) [110]. Similarly, a pooled analysis of 300 patients with recurrent glioblastoma treated in 8 phase I and II trials conducted by the EORTC brain tumor group found no association of repeat surgery with survival [111]. However, no stratified analyses and annotation of clinical predictors of survival, additional therapies or tumor volume were included in these reports.
The randomized controlled DIRECTOR trial, designed to explore the efficacy of two different dose-intensified temozolomide regimens at primary recurrence after standard first-line chemoradiotherapy, included 72 out of 105 randomized patients that underwent surgery at primary recurrence in 16 neurosurgical centers. No difference in the efficacy of both temozolomide regimens at recurrence was noted, thus providing a homogenous cohort that was well suited for the assessment of potential benefit from neurosurgery in subgroups of patients in a well-controlled setting [87]. In line with previous prospective datasets, surgery per se was not linked to improved post-recurrence survival (P = 0.633). However, post-recurrence survival was almost twofold longer in patients with complete resection of contrast-enhancing tumor (12.9 months, 95% CI 11.5–18.2), compared to patients with incomplete tumor resection (6.5 months, 95% CI 3.6–9.9), yielding a hazard ratio of 0.42 (95% CI 0.21–0.85) in a survival model adjusting for age, MGMT promoter methylation status, Karnofsky performance score (KPS), and steroid use at study entry. Despite the relatively small number of patients included in this trial, a strong trend toward inferior outcome upon incomplete resection became apparent, compared to patients not undergoing surgery (6.5 vs. 9.8 months, P = 0.052), thus indicating that repeat surgery should only be offered to patients where total resection of contrast-enhancing tumor seems possible [112]. Validation of these results may be achieved by the phase II RESURGE trial, which will randomize a total of 120 patients with recurrent glioblastoma in which complete resection of contrast-enhancing tumor is deemed safely feasible to receive surgery prior to systemic therapy, or systemic therapy alone (NCT02394626). Generally, tumor resections should be followed by systemic therapies, because infiltrating tumor cells beyond the radiographic tumor margin will generally remain and can drive tumor progression rapidly.
Radiotherapy
Elderly patients with MGMT methylated glioblastoma do commonly not receive first-line radiotherapy, and therefore, hypofractionated radiotherapy is the first-choice treatment at recurrence in this population [77]. The role of repeat radiotherapy in the remainder population of glioblastoma patients is less clear, because randomized controlled trials are lacking and due to the risk of iatrogenic harm from radiation necrosis. Reports from retrospective data analyses and uncontrolled trials suggest some activity of repeat radiotherapy in glioblastoma, particularly in patients with smaller tumor volumes, younger age, better general condition, and an interval of at least 6–12 months after first radiotherapy [113–115]. Typical dosing schedules that have been used are in the range of 30–36 Gy in 2–3.5 Gy fractions, usually applied as stereotactic radiotherapy. Hypofractionated regimens are also used. Radiosurgery and proton irradiation are rarely performed in glioblastoma, in part because the high precision of dose application that is the key characteristic of these techniques does not match the requirements of a diffusely infiltrating disease process.
The combination of repeat radiotherapy with alkylating or anti-angiogenic agents is supported by little or no evidence [103]. Novel agents continue to be explored. In a phase II trial of 91 patients with recurrent glioblastoma randomized in a 2:1 fashion to receive either repeat radiotherapy in combination with a fusion protein designed to target the cell surface death receptor CD95 (APG101), or repeat radiotherapy alone, the adjunct of APG101 showed a strong trend toward prolonged post-recurrence survival when adjusting for tumor size (HR 0.60, 95% CI 0.36–1.01), and hypomethylation in the promoter region of the gene encoding the CD95 ligand CD95L was associated with improved response to APG101 (HR 0.19, 95% CI 0.06–0.58) [116]. Whether the drug will be further developed remains uncertain.
Chemotherapy
Most patients are treated with single agent systemic treatments at recurrence of glioblastoma. Options that are usually well tolerated include CCNU/lomustine, temozolomide, and bevacizumab [117]. The alkylating agent lomustine is commonly utilized as a control in clinical trials for recurrent glioblastoma. Oral administration at longer time intervals (90–110 mg/m2 every 6 weeks) is advantageous in patients with impaired general condition, but progression-free survival rates at 6 months do not exceed 19–25% in clinical trial populations [118, 119]. Re-challenge with temozolomide is associated with longer post-recurrence survival, particularly in patients with apparent benefit from first-line temozolomide after a temozolomide-free interval [120]. Such patients bare mostly tumors with hypermethylation of the MGMT promoter [84, 87]. As outlined in the above section on first-line chemotherapy, dose-intensified temozolomide regimens are obsolete since more toxicity, but no survival difference was noted in two phase III trials directly comparing standard and dose-intensified temozolomide regimens in newly diagnosed and recurrent glioblastoma [100, 101]. Alternative chemotherapy agents such as procarbazine and carboplatin have also been used in the treatment of patients with recurrent glioblastoma, but no randomized data are available to support the efficacy of these approaches, whereas severe adverse effects can accompany their application. Systemic approaches to recurrent glioblastoma that are still under debate are discussed further below.
Molecularly Targeted Therapies and Future Directions
The histology-based definition of glioblastoma of the WHO classification of 2007 [78] has been increasingly complemented by the assessment of molecular markers, including IDH, MGMT and TERT status, as discussed in more detail in Chaps. 2 and 3. In prospect of a more personalized treatment of glioblastoma, molecular markers will be included in the revised WHO classification of 2016. However, a major challenge of decoding mechanisms of resistance is posed by temporal and intratumoral heterogeneity [121, 122]. Tumor progression and particularly failure of initially effective therapy regimens are accompanied by molecular adaptions due to clonal selection, acquisition of additional genomic alterations, epigenetic adaptions, and alterations in gene expression. For example, temozolomide chemotherapy can induce a hypermutation phenotype [123], and radiotherapy of proneural glioblastoma can induce rapid changes toward a mesenchymal gene expression pattern [124]. Yet, compared to the profound molecular landscapes generated of newly diagnosed glioblastoma, knowledge of the molecular patterns that underly glioblastoma progression and therapy resistance is only marginal and requires further joint efforts of the scientific community in order to develop effective molecularly targeted therapeutic approaches with durable efficacy.
Targeting Aberrant Signaling Pathways
Initial molecularly targeted treatments of glioblastoma and other cancers utilized small molecules to inhibit aberrant signaling pathways. RTK are the key mediators of extracellular signal transduction in glioblastoma, as outlined in more detail in Chap. 9.
RTK contain an extracellular receptor domain, which induces a conformational change upon ligand binding to initiate intracellular adenosine-triphosphate (ATP)-dependent tyrosine phosphorylation signals. The biological functions of RTK overlap widely and the convergence of downstream signals as well as crucial roles of RTK in healthy tissues complicate the development of tumor-specific RTK inhibition. However, the RTK inhibitor imatinib, designed to specifically fit the ATP-binding pouch of a cancer-specific tyrosine kinase derived from the BCR–ABL fusion gene, was found to offer a well tolerated, highly effective treatment for chronic myeloic leukemia [125]. This provided the incentive to develop personalized drug treatment, in particular RTK inhibition, in many cancers including glioblastoma.
Small molecule inhibitors of the RTK EGFR yielded disappointing results in glioblastoma, but EGFR amplification was not monitored in these trials, and blood–brain barrier penetration of all tested compounds was suboptimal [126].
Inhibition of the downstream RTK convergence molecule mammalian target of rapamycine (mTOR) utilizing temsirolimus (CCI-779) raised expectations in an uncontrolled phase II trial of 65 patients with recurrent glioblastoma: The radiographic response rate was 36% and high levels of phosphorylation of the mTOR target S6-kinase was associated with radiographic response and improved survival upon treatment with temsirolimus [127]. However, these results were not followed by a controlled trial to further explore the efficacy of temsirolimus in recurrent glioblastoma and a more recent randomized controlled phase II study of temsirolimus as an adjunct to standard chemoradiotherapy in patients with newly diagnosed, MGMT unmethylated glioblastoma failed to demonstrate improved survival (HR 1.16, 95% CI 0.77–1.76) [128].
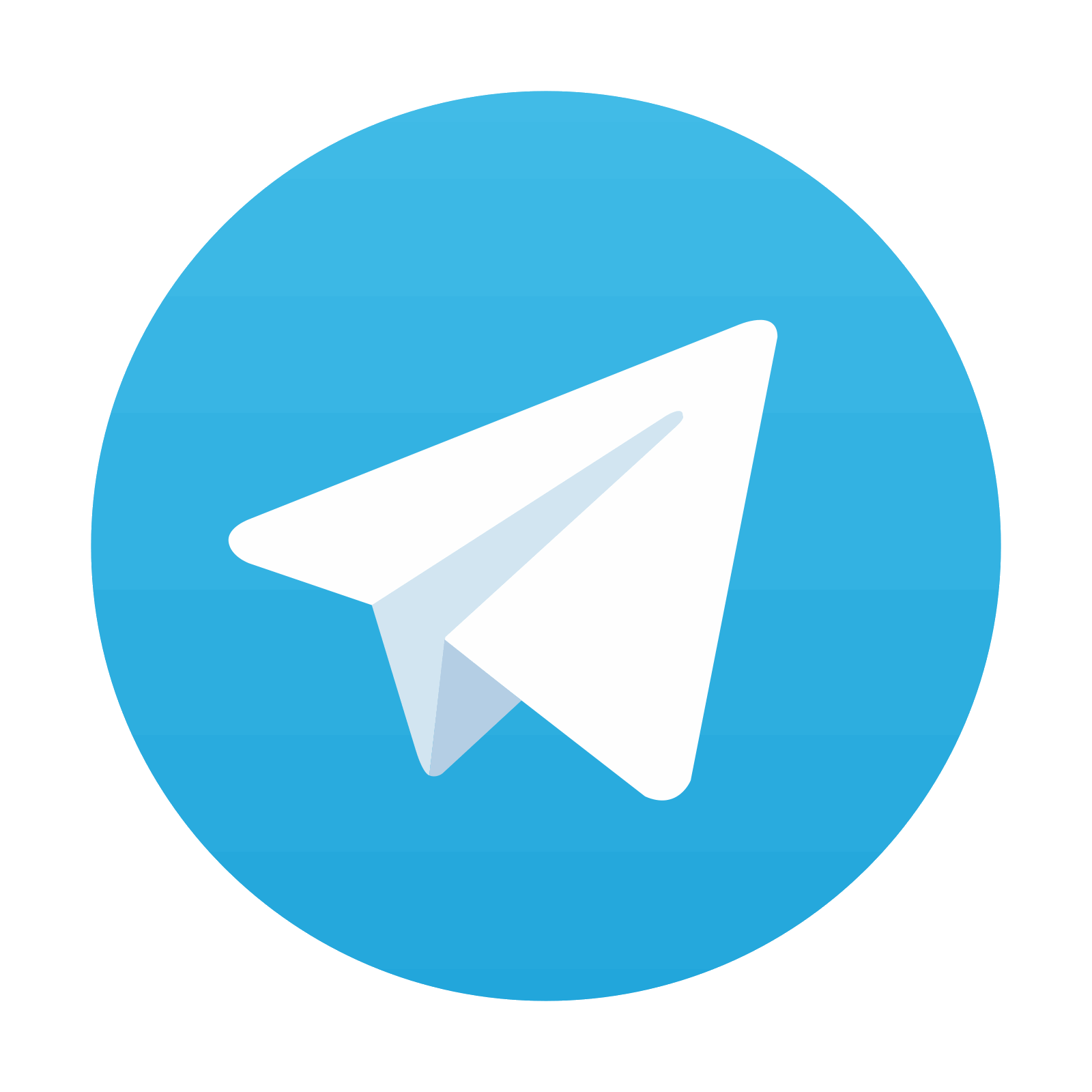
Stay updated, free articles. Join our Telegram channel
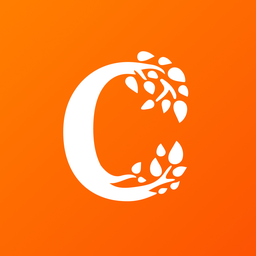
Full access? Get Clinical Tree
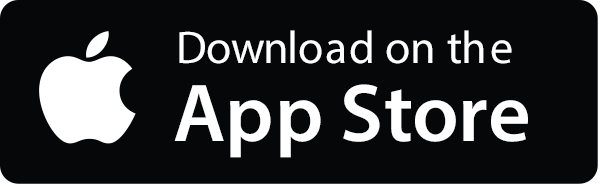
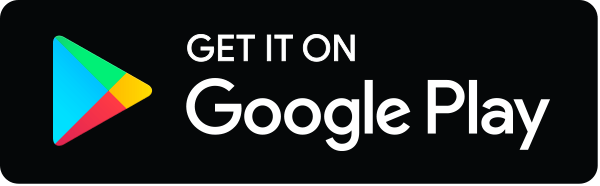