Abstract:
This chapter presents and discusses the integration of computer-assisted technology as one approach to maximize independence and quality of life in older adults and people with physical impairments. Rehabilitation robotics and computer-assisted technology use brain interfaces, sensorimotor interfaces, virtual reality environments, and learning-based gaming programs to remediate sensory, motor, and cognitive impairments and improve memory skills and physical abilities required for independent mobility and self-care at home, in the community, at work, and during the performance of recreational activities.
Keywords:
brain-machine interfaces, exoskeleton, human robotic interfaces, rehabilitation robotics, virtual reality training
Objectives
After reading this chapter the student or therapist will be able to:
- 1.
Summarize the need, demand, and principles for integrating advanced robotic technology in neurological rehabilitation.
- 2.
Define common terminology used in the field of rehabilitation robotics and technology.
- 3.
Classify the different types of advanced technology used in neurorehabilitation.
- 4.
Apply the guidelines for integrating robotics and assistive technology into a patient’s rehabilitation program.
- 5.
Discuss the benefits of performing a cost-effectiveness analysis when considering the application of robotic technology in rehabilitation.
- 6.
Describe the challenges of commercializing robotic devices.
- 7.
Discuss the future of advanced technology and rehabilitation.
Rehabilitation professionals face an ongoing challenge to provide clinically effective interventions that are also engaging and meaningful to the patient. The objective of rehabilitation technology is to empower clinicians and patients to take responsibility and control of the environment and facilitate physical and cognitive recovery. Technology assists patients during learning-based practice that drives neural adaptation and neural reorganization. For these reasons, robotic-assisted rehabilitation technology has been embraced by allied health and rehabilitation medicine over the previous two decades. It seeks to take interventions and dosages of therapy to the “next level”: a novel approach to offering high-volumes of computer/external force-guided body retraining.
Timely rehabilitation maximizes people’s performance of motor, speech, function, and cognition. The timing and purpose of introducing rehabilitation robotics and technology into the rehabilitation process will vary greatly. The “how” and “why” of robotic rehabilitation interventions need to be just as thoughtfully targeted as any other land-based approach for function, engagement, time-efficiency, and performance optimality.
Rehabilitation approaches across disciplines consider the influence of neuroplasticity on outcomes, and also consider the relationship of intervention to the World Health Organization’s International Classification of Functioning, Health and Disability (WHO-ICF). Whether the patient is an adult following a stroke or a child with cerebral palsy, the treating therapist should have a sound understanding of the principles of motor learning and neuroplasticity.
Furthermore, when reading this chapter, the clinician should reflect on the principles of experience-dependent plasticity ( Table 38.1 ) and align how robotic rehabilitation can augment each of these components for their patient.
Principle | Description |
---|---|
| Failure to drive specific brain functions can lead to functional degradation |
| Training that drives a specific brain function can lead to an enhancement of that function |
| The nature of the training experience dictates the nature of the plasticity |
| Induction of plasticity requires sufficient repetition |
| Induction of plasticity requires sufficient training intensity |
| Different forms of plasticity occur at different times during training |
| The training experience must be sufficiently salient to induce plasticity |
| Training-induced plasticity occurs more readily in younger brains |
| Plasticity in response to one training experience can enhance the acquisition of similar behaviors |
| Plasticity in response to one experience can interfere with the acquisition of other behaviors |
Robotic devices come in many shapes, sizes, and price points, which must be considered in selecting the best option. , For example, is it in the patient’s interest to have a wearable robotic device for everyday functional support? Is it more appropriate to access therapeutic bursts of complex, laboratory-based technology? There is no one answer, and the fine details of a patient’s therapy will be individualized. However, the path pursued by therapists alongside patients should be motivated predominantly by the patient’s own functional goals.
The first part of this chapter will provide an overview of the clinical utility of robotic technology in neurorehabilitation. As the field of robotic rehabilitation is dynamic, this section will not provide an exhaustive list of device options, nor will it provide explicit instructions on how to use devices. Interest in specific devices or approaches is best explored with the latest research outputs as they emerge and with the devices’ manufacturers directly. Instead, the aim of this chapter is to equip the rehabilitation professional with an awareness of how robotic and rehabilitation technology can be broadly applied to patients with neurological impairments and relate it back to the overarching principles of the WHO-ICF. Included in this part is the use of robotics for simulation for student education. The second part of this chapter will address other technology that is used in clinical and teaching environments, including inertia sensors, virtual reality (VR), and noninvasive brain stimulation.
Types of robotic rehabilitation and their applications
Robotic technology can provide service, body support, unweighting, and movement assistance that is passive, active, variable, and on-demand assistance. , Computerized and robotic technology provides the foundation for patients to practice and attend to purposeful, goal-oriented, progressive tasks spaced over time. This technology can also minimize the risk of injury during retraining. Robotic rehabilitation devices may include service robots, nonwearable assistive robotic devices, wearable assistive robotic devices, or neuromuscular stimulators. There are also vocational rehabilitation robotics, communication devices, and emotional interactive entertainment/friendly robots, which will not be investigated in depth in this chapter. Fig. 38.1 and Box 38.1 provide an overview of the classifications of robotics technology.

- •
Service robotics
- •
Assistive robotics
- •
Vocational robotics
- •
Prosthetic robotics
- •
Emotional robotics
- •
Communication robotics
- •
Virtual reality technology and robotics (including personal simulators)
- •
Learning-based gaming technology
- •
Technology for teaching
Service robots usually focus on task performance, movement assistance, and stability. These devices can be fixed, movable, or attached to a wheelchair. Assistive robotic devices help patients perform a task with direct or indirect assistance. Some of the assistive robotics are nonwearable but assist through unweighting or movement assistance. Wearable robotic devices are specifically designed to be worn by patients to assist movements. These are designed for the upper or lower limb. Vocational robotics can enhance performance at work either in terms of repetitive motions or high-force task production that would otherwise be dangerous to humans. Communication robotic devices are designed to improve communication potential for patients who cannot adequately speak or hear. Emotional support robotic devices are designed to provide emotional support for isolated individuals at home.
Prosthetics for amputees, vocational robotics, communication robotics, emotional support robotics, or socially assistive devices are not outlined in detail here as these areas are considered specialty oriented and may or may not be included in traditional neurorehabilitation programs coordinated by physical or occupational therapists.
For simplicity, we will focus on our examination on service robots, nonwearable assistive robotic devices, and wearable assistive robotic devices. It is also important to acknowledge there are a number of motorized chairs, lifts, and walkers available that can be used to transition a patient from sitting to standing, or provide unweighting while walking, or working on balance. These devices are not usually programmable and are not classified as “rehabilitation robotics” or “advanced technology.” However, these types of devices are very beneficial for helping patients maintain walking and training to improve safety and quality of gait at home and with supervision. It is important for therapists to be sure these types of assistive devices have been considered and/or integrated into a patient’s rehabilitation program and at home before recommending more sophisticated technology.
Service robotics
Service robots primarily assist individuals with severe disabilities. Most commonly, the robot performs everyday activities (e.g., assisting with eating, drinking, object replacing, ambulating). There are three main types of schemes: desktop-mounted robots, wheelchair-mounted robots, and mobile autonomous robots. In general, these robots are used in the home, are interconnected to a variety of control systems, are programmed to the environment and consequently are not very portable.
These types of robotic devices are often preprogrammed to perform certain tasks. There are also some autonomous robots in which the cognitive interface between the user and the robot is used to tell the robot to perform a new task or to help the patient perform the task. These robotic systems are successful if the robot, the user, and the manipulated objects remain in the same initial set position every time a concrete task is performed. With the wheelchair-mounted manipulator, the relative position of the user with respect to the manipulator needs to remain the same. Although there are a variety of simple service-based robotic devices, most are complex, and setting them up at home generally requires a computer or engineering specialist.
Service robots may include smart wheelchairs and smart devices in the home (e.g., The Robotic Room ) or wheelchair-mounted upper extremity manipulators. A major consideration for patients using service robotic devices are the control options. For example, through the use of headpieces on robotic devices, information can be detected from flexion and extension, rotation, and side bending of the head to operate wheelchairs, TV sets, telephones, doors, and security systems. There are also interfaces that are sensitive to facial movements and optoelectronic detection of light-reflective head movements. Other interfaces are sensitive to eye movements or use voice recognition, brain control, , and gesture recognition. These interfaces not only may allow control of the robot but also may be applied to move a limb or perform a task. A more detailed discussion of robotic interfaces is included later in this section.
Service robotics is recommended when patients have achieved their maximum potential and still need assistance to live independently. A therapist may continue to work with a patient at home to maintain range of motion, minimize skin problems, and review whether the robotic technology is still providing the necessary assistance. However, an engineer will usually assume the primary responsibility for maintaining and adjusting the robotic equipment.
Nonwearable assistive robotic devices
This group of robotic devices primarily includes powered wheelchairs with autonomous intelligence, robots for body support, and hands-off service robotic devices. Body weight supported treadmill systems (BWSTSs) with and without robotics and body weight-support mobile walking aids (BWSMWA) are also aligned to this category. , The value of BWSTSs and similar nonwearable assistive robotic devices is that patients can be supported to develop their body structures and functions (e.g., step length, weight shift abilities, balance) as a precursor to practicing tasks at the activity/participation level in more authentic, natural settings. Body weight–supported gait training systems were initially designed to unweight the body, decrease ground reaction forces (GRFs), and protect against falling ( Fig. 38.2 ). Under these assisted conditions, it is easier for patients to achieve intense levels of exercise such as walking, skipping, and running with less pain and less trauma to the joints.

Assistive robotic devices hold a prominent place in the rehabilitation of adults and children with neurological dysfunction. , Robotic-assisted BWSTT has been investigated for individuals with chronic spinal cord injury and stroke. Evidence of greatest efficacy of these robotic or electromechanical-assisted devices has been reported in the first 3 months following stroke, and of most benefit to those with very limited walking ability. Furthermore, it is important to support patients to walk at community-level velocities of at least 0.8 m/s (faster than 2.0 mph). Devices such as the Lokomat, which robotically augment joint placement and walking execution on a treadmill, have been examined in children with neurological impairments. Children with cerebral palsy may experience greatest benefit when they have moderate-to-severe degrees of gait impairment (e.g., GMFCS levels II, III, and IV). The opportunity for clinically noticeable training change is greatest and more likely to achieve physiological and possibly neuroprotective benefits. ,
Nonwearable assistive robotic devices can offer patients the potential for high degrees of repetitive practice by adjusting, placing, and guiding the limb through repeated movements. , , It can help reduce the taxing physical effect and awkward positioning of rehabilitation on clinicians while offering even higher volumes of practice in a single session. However, at some point, clinicians and patients must face the possibility of a ceiling effect of high-dose practice in a predictable environment. The therapeutic course should take the patient to eventually progress to task-specific training outside of assistive robotic devices, which is where conventional approaches to therapy take precedence.
Wearable assistive robotic devices
Assistive robotic devices are also popular in upper limb rehabilitation. The upper limb is highly complex, with a requirement for devices to be appropriately light and responsive to degrees of movement. The shoulder and hand/wrist will demand multidimensional options compared to the elbow. This becomes challenging for designers to balance aligning the device with joints, as well as incorporating motors, actuators, and cabling. The clinician will therefore decide whether the patient requires an exoskeleton device (e.g., Armeo) with torque actuators that control single/multiple joints, or an end-effector-type device (e.g., InMotion 2.0), which focuses on the distal portion of the upper limb. A recent study on post-stroke intervention notes a higher degree of exploration of end-effector devices as newer exoskeleton technologies have been less commonly investigated. It also notes that meaningful change, especially with earlier intervention, will be assisted with some degree of voluntary muscle initiation of wrist and fingers; timing of intervention remains an important consideration.
Wearable assistive robotic devices can support patients to participate in everyday tasks with greater autonomy. Structures may be exoskeleton but with rigid or soft wearable features. They may be fixed in place in a clinical training station or they may allow great portability with movement and use. Examples of these include the ironHand system or those that are triggered with neuromuscular stimulation, such as the Bioness upper limb or lower limb systems (see Fig. 38.3 ). The primary impairment that can be addressed with neuromuscular stimulation is muscle weakness, but the patient may also have neuromotor control problems or abnormal synergies of movement. These devices work only when peripheral nervous system function is preserved. The electrodes, located within the device’s control unit, stimulate a peripheral nerve causing a movement effect. However, in the case of patients with spinal cord injuries, multiple electrodes may need to be used to sequentially activate a series of muscle contractions to enable walking. Similar robotic devices and principles of operation are available for patients post-stroke.




Wearable assistive robotic technology extends, complements, empowers, replaces, or enhances the function or capability of the individual. For some patients, using these is an ongoing component of their rehabilitation. For others, the wearable assistive robotic device is used as it can complete the action of a task that is no longer an effective or efficient option for the patient. These robotic devices may facilitate muscle contractions in one or more directions around one joint such as the elbow, wrist, or ankle. The objectives of reducing muscular fatigue and energy conservation during task practice are met when devices are lightweight, flexible, sensitive to movement change, and easily portable. , ,
Upper limb and lower limb wearable robots are obviously worn by the individual, but the internal or external interface must be mapped to the anatomy, a cognitive control mechanism, or the brain. The extremities can be used to perform multiple tasks, and the redundancy of the joints and degrees of freedom (e.g., at the ankle, or the wrist) allow people to perform tasks in a variety of ways. Redundancy of the joints and degrees of freedom allow a variety of ways patients can perform a task; however, these redundancies may be hard to control. Wearable robotic devices will vary by type of human interface system incorporated (e.g., brain neurons, cognitive, sensory [tactile, pressure], physical [movement], or breath). At a minimum, the physical interface of a wearable robot requires an actuator and a rigid structure to transmit forces to the neuromusculoskeletal system.
Ideally, wearable systems use both muscle stimulation and active voluntary muscle contractions to increase functional use. Devices such as the ReWalk and the Hybrid Assistive Limb (HAL) are well known commercially, and among clinicians working in neurological rehabilitation, for their wearable design and capacity to support patients with spinal cord injuries particularly, to ambulate with relative independence. With actuation componentry at the hip and knee, these devices facilitate gait with electromyographic triggered stimuli (HAL) or battery powered, remote-control operation mechanisms (ReWalk). As with the upper limb orthoses though, wearable exoskeleton technologies guiding lower limbs have key limitations around their size, bulk, weight, and responsiveness to the external environment. There can be some limitations in transferring the device usage into more complicated, unpredictable terrains. However, as a concept, wearable lower limb exoskeletons such as the ReWalk and HAL can have significant influence at the activity and participation level. If accessible to rehabilitation staff, they may be worthy of consideration for goals that align to these dimensions of the WHO-ICF. Other device examples recently cited in the literature include Ekso exoskeleton, REX Personal, and the Indego exoskeleton.
Robotic system mechanisms for operation
Robotic interfaces, actuators, and controllers can convert sensory, physical, and cognitive signals to:
- •
control robots
- •
permit perception of spatial relationships
- •
mobilize individuals in space
- •
assist in object manipulation
- •
provide emotional support
- •
allow individuals to call for help and communicate with others
In addition, through creative virtual training environments and gaming technology, patients can improve memory, motor skills, and movement quality.
Different robotic designs enable different performance outcomes. Understanding robotic mechanisms of operation is an important first step for a clinician as it may guide the selection of devices for patients. Robotic devices in general may be active, passive, haptic, or predominantly performance feedback-oriented. , The degree of assistance offered will determine the suitability of robotic mechanical design, and vice-versa. Maciejasz and colleagues best summarized different robotic systems by feature, outlined in Table 38.2 .
Term | Description |
---|---|
Exoskeleton devices | These use an externally-fitted framework to replicate the targeted limb/limbs’ skeletal structure. The limb’s joints are aligned with the framework’s joints to support movement. An exoskeleton device may replicate multiple joint movements, but also can be set up to both assist and inhibit body segment use |
End-effector-based devices | Usually simpler than a full exoskeleton device, end-effector designs have a single, distal point where mechanical forces are applied to the distal limb segment. Note that the simplicity of the design may offer challenges when the limb position demands multiple degrees of freedom |
Back-drivable devices | A feature of robotic design where the patient can move the device without robotic augmentation. Importantly, limb movement is not constrained while fitted to the device |
Planar robotic devices | These devices operate in a specific plane of movement and are often associated with end effector designs |
Modularity | A property of a device indicating that optional parts may adapt it to a specific condition or simply to perform additional exercises |
Reconfigurability | A property of a device indicating that it mechanical structure may be modified without adding additional parts in order to adapt it to the condition of the subject or to perform other forms of training |
The robotic device’s mechanical design (e.g., exoskeleton) also has specific properties, which may require some understanding. These properties can help determine the degree of augmented movement available in the device, , as outlined in Table 38.3 .
Term | Description |
---|---|
Electric actuators | Actuators powered by electric current. They are the most common because they easily provide a relatively high power and are able to store energy. There is a wide selection of commercially available electric actuators; however, some of them are heavy and/or their impedance is too high for rehabilitation settings |
Hydraulic actuators | Actuators powered by hydraulic pressure (usually oil). They are able to generate high forces. Their system is relatively complex considering the maintenance of pressurized oil under pressure to prevent leakage. Commercial hydraulic actuators are also heavy; therefore only specially designed hydraulic actuators are used in rehabilitation robotics |
Pneumatic actuators | Actuators powered by compressed air. They have lower impedance and weigh less than electric actuators. Special compressors or containers with compressed air are required for power |
Pneumatic Artificial Muscle (PAM, McKibben type actuator) | A special type of pneumatic actuator with an internal bladder surrounded by a braided mesh shell with flexible, but nonextensible threads. Because of their specific design, an actuator under pressure shortens, similarly to the contracting muscle. It is relatively light and exerts force in a single direction. It is difficult to control because of its slow and nonlinear dynamic functions |
Series Elastic Actuator (SEA) | A generic name used for a mechanism with an elastic element placed in series with an actuator. This solution is relatively often met in the design of rehabilitation robots. It decreases the inertia and intrinsic impedance of the actuator to allow a more accurate and stable force control and increase patient safety |
Functional Electrical Stimulation (FES) | It is a technique that uses electrical current to activate nerves and contract their innervated muscles. It produces the movement of the limb using natural actuators of the body. However, it is difficult to achieve precise and repeatable movement using this technique, and it may be painful for the patient |
Clinical rationale for the use of robotic devices is specific to the patient and the particular device. Robotic devices can be used to improve body structure and function, activities, and participation. For example, the Lokomat (see Fig. 38.2 ) uses a body weight–supporting exoskeleton and provides augmented limb movement to improve the functions of weight-bearing tolerance and sagittal plane joint mobility. , Other devices, such as the ReWalk ( Fig. 38.4 ), support the spinal cord-compromised patient to ambulate with crutches, allowing community mobility and potentially greater autonomy ; performance factors that align with “participation” expectations. Patients in early rehabilitation phases may respond better to a particular type of robotic device, superseded by an alternative option as the patient’s capabilities evolve or diminish. There also may be changes between genuine neurological structure recovery (e.g., CNS reactivation) and the emergence of effective compensatory strategies for movement. Iosa and colleagues have recently proposed the “ideal” interactional loop between the patient, the rehabilitation professional, and the robotic rehabilitation device selected ( Fig. 38.5 ).


Aligning the device category, actuation componentry, design, and function to the patient’s needs can confidently provide the clinician with a plan for robotic application. Many therapists will be limited by what their workplace has access to.
General screening considerations
There is a potential pitfall for patients and therapists to pursue technology for “technology’s sake.” A robotic device does not replace an individualized, task-specific, land-based regime and should not be a substitute for goal-oriented therapy. Importantly, the patient and therapist should not become tempted to force the robotic options on offer to become a part of the patient’s therapy if it does not align with functional goals. Box 38.2 summarizes the principles to support the use of technology and robotics in therapy.
Principle I
Goals for advanced technology and rehabilitation robotics include the following:
- A.
Indirectly augmenting functional independence of individuals with impairments by:
- 1.
performing mobility tasks for individuals at the home (e.g., using automatic motorized wheelchairs to move individuals from room to room; transitioning individuals from bed to chair and from chair to standing; moving patients who are standing; smart houses; calling for help)
- 2.
minimizing the need for assistance from another individual
- 3.
performing functional activities of daily living (activities of daily living; e.g., getting objects, cooking food, doing dishes, bathing, transferring)
- 4.
helping perform difficult or repetitive tasks at work (e.g., assembly line tasks; lifting and moving heavy objects)
- 1.
- B.
Directly improving human motor skill capabilities of individuals with impairments to enable them to:
- 1.
perform functional tasks independently
- 2.
improve voluntary control
- 3.
perfect quality of movement
- 4.
learn new skills
- 1.
Principle II
Advanced technology and rehabilitation robotics should maximize neural adaptation and reorganization through the creation of practice opportunities that:
- A.
are attended, repetitive, purposeful, goal oriented, progressive, and spaced over time
- B.
are fun, interesting, and practical
- C.
are task oriented
- D.
provide feedback on accuracy of task completion
- E.
can be matched to patient abilities
Principle III
The objectives of assistive rehabilitation robotic devices need to be clearly defined in terms of:
- A.
unweighting a limb to reduce patient effort required for movement
- B.
actively canceling mechanical limitations on movement of the patient and robot arm dynamics (e.g., friction, inertia, and weight under gravity)
- C.
gently and progressively moving a limb to assist patient effort to perform a task
- D.
stabilizing a joint to enable a patient to produce a controlled movement
- E.
assisting the patient to improve the accuracy and quality of a movement
- F.
assisting the sequencing of movements
- G.
providing resistance to strengthen movements
Principle IV
Robotic technology must be:
- A.
safe for training
- B.
reliable in performance
- C.
able to reduce risks of injury (e.g., falls)
- D.
able to minimize injury during use
Principle V
Robotic technology should be:
- A.
adaptable (across patient needs, side, type of actuation)
- B.
able to integrate interfaces, actuators, and controllers sensitive to the ability of the individual
Principle VI
Robotic devices need to be:
- A.
reasonably priced and cost-effective
- B.
versatile
- C.
durable
- D.
repairable
- E.
easy to use
Principle VII
Wearable robotic devices must be:
- A.
lightweight
- B.
easy to get on and off
- C.
portable
- D.
cosmetically acceptable
- E.
interfaced to patient ability (sensory, motor, cognitive, brain)
- F.
minimally harmful to the skin
- G.
dynamically adaptable to performance capabilities
Principle VIII
Robotic technology for rehabilitation needs to be defined by:
- A.
location of the control system relative to the patient (controlled at a distance from the user [e.g., Web, Skype]), controlled in proximity to the user [e.g., by a therapist or engineer], or controlled by the user [e.g., wearable device or interface])
- B.
environmental connection of the device and the patient (fixed to a nonmobile surface [e.g., wall], attached to a mobile platform [e.g., wheelchair], freely mobile with the patient [wearable])
- C.
type of control system (e.g., joystick, sensor, breath)
- D.
type of interface (physical, sensory, cognitive, brain)
- E.
type of anatomical connection (e.g., by end effector only, end effector and multiple points of attachments with serial links or temporal links)
Principle IX
Brain controlled interface rehabilitation robotics must be:
- A.
considered after all other alternatives have been unsuccessful
- B.
minimally intrusive despite surgery
- C.
physically accessible via remote control outside the brain
- D.
controlled with minimal patient risk and with safety
- E.
potentially controlled by cognition or the patient’s mental effort
- F.
able to extend patient’s independent task performance
The criteria used to determine which patients might benefit from rehabilitative technology will change over time. Devices will come with specific instructions and suggestions for use/contraindications to use. However, the treating therapist must also make an informed decision to use the robotic technology because it holds promise to “value-add” to the patient’s performance. Before screening a patient for robotic technology, clinicians need to be certain that all standard assistive devices have already been integrated into the individual’s rehabilitation program. Ultimately, guidelines should be developed to match the potential of the individual with the prognosis for independence, with and without dependable, user-friendly technology.
An objective evaluation is needed to match a patient with a commercially available robotic device. This evaluation must include a thorough assessment of anatomical, physiological, cognitive, and sensory impairments. Whenever possible, standardized tests should be used to document strength, flexibility, endurance, balance, coordination, synergistic responses, hypertonicity, gait, balance, posture, and postural righting skills. These impairments need to be integrated into functional and task-specific assessments of motor learning, motor control, activities of daily living (ADLs), work requirements, and recreational needs. Then each patient should be screened by defined objectives relative to outcomes in terms of quality of life and independence. Subjective and emotional issues such as attention, motivation, history of positive health behaviors, durability, depression, desire for independence, and commitment to learning must also be considered. As an additional consideration for children, the parents and caregivers must confidently understand the value and purpose of including the device in a therapy regime and the therapist must feel comfortable that the child can express discomfort or displeasure with the device, to minimize adverse effects. There is evidence to suggest that with devices such as the Armeo and LokomatPro, for example, the more motivated to avatar-based games the child is, the greater the clinical effects. , Speculation is that active involvement in gaming will increase the responsiveness of body structures and functions (e.g., postural control, weight shift) to gait rather than the passivity that comes with walking on a treadmill engaging in less-interactive watching of movies on screens. Indeed, recovery for patients may even be compromised if tasks are monotonous, demand less effort or focus.
The patient needs to go through general and specific screenings to determine the appropriateness of integrating a nonwearable assistive robotic device into the rehabilitation program ( Table 38.4) . The patient who is most likely to benefit from body weight–supported gait training is the patient who has the prognosis for functional independence but needs to train to improve quality, endurance, speed, and stability of gait. Thus many patients with problems described within this text might benefit from this type of training. Those who do not have the potential for independent ambulation could still benefit from training on a BWSTS. In these cases, the training would be directed toward enhancing metabolic health, providing a sense of well-being, increasing circulation, and minimizing secondary impairments associated with excessive sitting such as decubitus ulcers and bone demineralization. These patients may need robotic, human, or harness assistance to achieve standing or stepping as well as bracing of the neck and trunk when upright. Patients with joint pain in the back or knee may also benefit from wearing supports when standing or exercising.
ASSISTIVE ROBOTIC DEVICES | |||
---|---|---|---|
Screening Criteria | Service Robotic Devices | Nonwearable—Mobility and Unweighting | Wearable—Mobility and Object Manipulation |
Criteria for determining potential benefit for patient to integrate advanced or robotic technology | Patient with severe physical impairments challenged to be independent without personal assistance:
| Patient with mobility impairments compromising safety, full independence, and quality of life:
| Patient with impairments compromising mobility and/or independent task performance at home, at work, or during recreation:
|
Screening criteria to determine if patient has the ability to use advanced or robotic technology | Patient has potential to achieve independent wheelchair mobility and ADLs at home with reduced human assistance:
| Patient has the potential to improve walking quality and speed, endurance, and independence with progressive practice or training:
| Patient has the sensory, physical, and cognitive ability to control a wearable device to achieve independence in mobility and/or object manipulation:
|
Screening criteria to determine temporary versus permanent need for technology | Patient has:
| Patient has:
| Patient has:
|
Screening criteria to determine safety | Patient has:
| Patient can:
| Patient has:
|
Screening criteria to determine accessibility of advanced or robotic technology | Patient has:
| Patient has:
| Patient has:
|
Screening criteria to determine cost-effectiveness of advanced technology or rehabilitation robotics | There are clinical research trials reporting:
|
|
|
If a patient does not demonstrate the ability to bear weight on a single limb, then robotic control of the lower limbs may be the preferred mechanism to facilitate secondary benefits. However, when all the movements are passive, there will be minimal gains in terms of neural adaptation and reorganization. On the other hand, it may counter the secondary problems of bone demineralization and decubitus ulcers and be of value as a maintenance strategy.
In spite of advancements in rehabilitation robotic technology, some patients may still have difficulty taking advantage of this type of therapeutic assistance. In patients with challenging impairments, for safety the use of an overhead harness to access the treadmill may be required, in addition to close supervision by one or more therapists.
Specific screening of patients for wearable assistive devices
Screening must be sensitive to the characteristics of the individual and consistent with factors in Table 38.4 . After identifying the parameters of performance and the potential benefits of assistive wearable technology, the patient, the family, the therapist, and sometimes the orthotist should determine what assistive robotic devices or advanced technological equipment are available to meet the patient’s needs. The team also has to assess where the devices are located, whether they are accessible, whether the device should be rented or purchased, and whether the insurance company will help pay for the rental or purchase of the device.
Ideally patients will use a wearable robotic assistive device to try to drive neural adaptation to recover function. Thus some patients may “train out” of the robotic device as they recover more function. Obviously, it would be better for these patients to rent rather than purchase the orthotic. Other patients would benefit from purchasing the assistive device because the robotic device improves function and independence despite ongoing impairments. Long-term use is also common when a patient has a degenerative condition, when the impairments are likely to get worse rather than better, and when with assistance independence can be prolonged.
In addition to the general and specific screening criteria, it is important to note that some assistive robotic devices may target control of one specific joint. However, given the biomechanical links, the flexibility, sensory, and motor characteristics must be assessed at each major joint above and below the primary assisted joint. The less affected side also needs to be evaluated. To maximize the benefit of a wearable assistive device, patients should ideally have some ability to voluntarily initiate movement and a grade of poor or greater strength to be able to assist in the movement.
Wearable robotics must be programmed not only to assist patient function but also to stop to avoid harm. This requires a balance between the dynamic nature of the wearable assistive device and a patient’s weakness, lack of voluntary motor control, and the presence of involuntary muscle activity including hypertonicity (e.g., spasticity, dystonia, rigidity, tremor). For example, how much force would the assistive device need to provide to overcome involuntary tone? Other relevant questions must then be asked: If a robotic device is programmed to assist with flexion and the patient initiates movement into extension or abduction, will the robot have to stop assisting the limb to prevent harm? If the robot stops assisting, is there a negative effect caused by the mismatch of force between the patient and the robot? Does the patient need consistent assistance or variable assistance? How will the therapist, the robot, or the patient determine how much assistance is needed? In one case a patient may need unweighting of only a limb, and in another case, both unweighting and assistance may be needed. In theory, the amount of assistance needed should decrease with recovery of function. It is helpful if the wearable assistive device can easily be adjusted by the therapist or the patient.
Despite the advancements in rehabilitation robotics, some patients will still have difficulty wearing an assistive orthotic. For example, wearable assistive orthotic devices may not work well for patients with severe sensory impairments, severe balance problems, a fear of falling, inadequate assets to control the device or for elderly patients who are afraid of computerized technology. Sophisticated rehabilitation robotic devices ( Boxes 38.3 and 38.4 ) also may not be recommended for patients who are disoriented, who have severe pain or neural hypersensitivity, or who cannot don the apparatus independently.
- •
Fixed—preprogrammed task performance
- •
Mobile service—mechanical slaves
- •
Wheelchair-mounted—upper-limb manipulators
- •
Automatically guided wheelchairs
- •
“Smart houses”—designed for independence
- •
Body weight–supported mobile walking aids
- •
Robotic devices for physical support (indoors, outdoors)
- •
Robotic devices for physical support, unweighting, and mechanical stepping in place
- •
Robotic devices for unweighting and controlled destabilization
- •
Robotic devices for unweighting and gait training
- •
Robotic devices for unweighting and robotic stepping on a treadmill
It may be appropriate and helpful for patients with severe sensory and motor impairments to train using VR technology with or without assistive technology. Patients may begin with mental imagery and practice before engaging in physical practice, without and then with the integration of wearable robotic devices. It is also possible to begin the training with the assistive technology while in a harness system to protect the patient from falling. In addition, depending on the severity of balance and voluntary abilities, it may be necessary for patients to train with technology under careful direct supervision. In cases in which balance and motor control are good but can be improved, it may be possible for patients to train at home with wireless telemetry-type supervision.
Please refer to the Evolve site for a video case illustrating the use of technology in the rehabilitation of a child with cerebral palsy.
Patient simulator robotics
Purpose
One area of robotics contributing to improved rehabilitative care and education of rehabilitation professionals is the development of programmable human patient simulators. This section of the chapter describes programmable human patient simulators that are currently available. Programmable human patient simulators can be used to replicate many common medical conditions. Simulation is used to train clinicians so that when those rehabilitation professionals encounter a similar clinical situation they can respond more efficiently and effectively.
Introduction
Simulation using task trainers, actors, and standardized patients is not new to the education of rehabilitation professionals. While task trainers are useful for development of specific skills (e.g., cardiopulmonary resuscitation [CPR]), immersive simulation using high-fidelity robotics can be used to simulate and practice working with a variety of medical conditions, situations, and health care personnel. In addition, these scenarios can be repeated so every student has an opportunity to deal with a variety of specific medical conditions including rare but critical events (e.g., acute myocardial infarction [MI]).
In 2011 Stockert and Brady reported that approximately 44% of entry-level physical therapist professional education programs in the United States were using some form of programmable human patient simulator. In 2017 Stockert and Ohtake reported that the percentage of entry-level physical therapist professional education programs using programmable human patient simulators for immersive simulation had increased to 70% and about 40% of those programs were using the technology for interprofessional education (IPE). , Education programs reported using the technology to train health care providers from multiple disciplines for interprofessional team responses to the challenges of providing care for a patient suffering an acute MI or a patient in need of early mobility in the intensive care unit. About 90% of the programs using simulation for IPE reported including the IPE Collaborative learning objectives for interprofessional, collaborative practice. , The vast majority of these programs included interprofessional teams of de-briefers consistent with best practice patterns described in the literature. The authors noted that they anticipate a further increase in the utilization of immersive simulation as an educational strategy for IPE in order to promote interprofessional collaborative practice and to comply with recently revised accreditation standards related to IPE in multiple health care professions.
Currently available robotic patient simulators can be programmed to demonstrate clinical signs and symptoms consistent with a diverse group of clinical conditions seen by Physical Therapists and other rehabilitation professionals. The patient simulator can be programmed to present signs and symptoms that require a participant to analyze and determine the urgency of the situation, assess the “patient” status, determine an appropriate intervention, and see the consequences of their action or inaction without compromising patient safety. A wide variety of clinical conditions and situations can be presented in an effort to ensure that each participant has an opportunity to practice and receive feedback regarding their clinical performance with multiple medical conditions. Simulation experience is critical for practicing how to respond to common as well as rare but critical events (e.g., acute MI). The learner’s ability to respond appropriately during a simulated event can increase the likelihood that those rehabilitation professionals are adequately prepared to respond efficiently and effectively when encountering a similar situation in the clinic.
Technology
At the beginning of 2018 there were two primary manufacturers of programmable human patient simulators. METI Learning (Medical Education Technologies, Inc.) started making patient simulators in 1996 and was acquired by CAE Healthcare in 2011 ( https://caehealthcare.com/ ). Asmund Laerdal, with Drs. Lind and Safar, developed “Resusci Anne” for learning CPR in 1960. The Laerdal Corporation has been making a variety of patient simulators since that time ( https://www.laerdal.com/us/ ). In this chapter on rehabilitation robotics, the properties and capacities of programmable human patient simulators are discussed based on currently available models by those two manufacturers. This analysis will hopefully help rehabilitation professionals evaluate the benefits of new robotic simulators for professional education.
Features
The number of features available on programmable human patient simulators continues to increase and raise the degree of realism (fidelity) attainable during immersive simulation. Many features, including eyes that blink and the simulated patient conversing in real-time, make the clinical scenario realistic for the participant. Several clinically relevant characteristics of human patient simulators are described on the following pages.
Size.
The size of the adult human programmable patient simulator mannequins is similar to an average-sized full-grown adult, but the weight is significantly less than average (approximately 85 pounds). In addition to adult models, there are baby simulators, child simulators, and birthing simulators. Some models are wireless. This discussion focuses on the features and properties of the adult high-fidelity simulators available in 2018.
Limb and chest mobility.
The upper and lower extremities of most programmable patient simulator models have very limited passive mobility with the exception of the shoulders, hips, knees, and ankles, which approach “normal” for an adult. The limited mobility of the limbs allows for some range of motion and bed exercises to be simulated. However, clinicians will note the completely passive, and somewhat awkward, nature of the movement and that the limb weight is significantly less than normal.
The chest wall moves realistically during ventilation. The excursion of the chest can be programmed to be symmetrical or asymmetrical in order to mimic specific clinical conditions. In addition, the rate and depth of ventilation can be manipulated during the course of a simulation.
Physiological features.
High-fidelity programmable human patient simulators have realistic physiological features. A library of normal and abnormal breath sounds, heart tones, bowel sounds, and other sounds is available to aid in simulating a variety of clinical conditions. All of the breath sounds, heart tones, etc., can be changed during the course of a simulation to reflect a change in “patient” status during a scenario.
The simulation mannequins can be connected to multiple lines and tubes to simulate an acute care and/or critical care environment; for example, arterial line, intravenous line, nasogastric tube, electrocardiograph wires, and nasal cannula. Lines and tubes, appropriate for the simulated condition, add to the realism of the scenario. The presence of multiple lines and tubes allow the participant to practice identifying the lines and tubes, verify the patient status, and practice “clearing” the lines.
A patient monitor, similar to those found in critical care settings, is part of the setup to enhance realism. The monitor can be customized to display a number of different physiological variables including arterial blood pressure, pulmonary blood pressure, respiratory rate, cardiac rhythm, hemoglobin saturation, intracranial pressure, pH, temperature, and others. The value and number of variables shown on the monitor provide real-time patient data and can be programmed to change during the course of the simulation to simulate clinical conditions and/or a change in patient status.
Pulses are palpable in a variety of locations including the carotid, brachial, radial, femoral, popliteal, and dorsalis pedis pulses. The pulse is synchronized with the electrocardiogram (ECG), and the pulse strength can be varied during a simulation. When a simulation participant palpates the pulse, a notation is made in the computer log that specifies which pulse was palpated and at what point in time during the simulation the pulse was taken. In addition, when blood pressure is low, some pulses (e.g., the dorsalis pedis) are no longer palpable, simulating the clinical condition of systemic hypotension.
High-fidelity human patient simulators are capable of vocalizations using a variety of sounds stored in the simulation computer. However, the simulation operator is able to speak into a microphone and/or use audio files to produce sounds that appear to come from the mouth of the mannequin where a speaker is located. The capacity to converse in real-time greatly elevates the fidelity of the scenario.
Cyanosis is simulated through the use of a light contained within the mouth that makes the lips and gums appear blue. The eyes can form tears, the eyelids blink, and the pupils are reactive to light. The skin in the forehead is capable of diaphoresis. In some areas, the skin has “wound modules” that can be inserted and connected to ports that contain “blood” used to simulate bleeding from the wound site.
Cerebrovascular system features.
High-fidelity human patient simulators can be programmed to display signs and symptoms consistent with an acute cerebrovascular accident (CVA) or traumatic brain injury (TBI). The simulator has the potential to display a slurred speech pattern, a drooping eyelid, asymmetrical pupils, and emotional lability. The simulator can state that their arm or leg feels heavy and that they cannot feel the limb. The patient monitor can be programmed to show a variety of changes consistent with an evolving CVA or TBI, such as changes in blood pressure, heart rate, intracranial pressure, and respiratory rate.
Cardiovascular and pulmonary system features.
The onset of an acute MI is a scenario commonly used in simulation to train clinicians. The patient simulator can be programmed to complain of chest pain while simultaneously developing diaphoresis. The patient monitor can show the changes in the blood pressure, heart rate, respiratory rate, and cardiac arrhythmias consistent with an acute MI. Scenarios can be used to practice recognizing the signs and symptoms related to multiple acute medical conditions as well as to practice interdisciplinary health care team responses to acute crises. The advanced features of the cardiovascular system include the capacity to bleed from a variety of portals. The system permits health care personnel to practice advanced cardiac life support (ACLS) protocols and to practice team responses to medical emergencies. The ACLS features built into the patient simulators measure and record the rate and depth of chest compressions, as well as hand placement, during CPR. This allows “coaching” of the participants’ CPR skills/technique in real-time.
The physiological response to a variety of drugs and clinical conditions is programmed into high-fidelity models. This feature allows participants to witness the response to the administration of medications as well as adverse drug reactions. The advanced clinical features for the pulmonary system include the ability to intubate (esophageal and endotracheal) and insert chest tubes into the mannequin, providing students and clinicians an opportunity to practice their clinical skills with “patients” who are intubated and/or have a chest tube. Some simulators allow the participant to practice pulmonary suctioning techniques.
A variety of cardiac arrhythmias are programmed into the patient simulators, and these can be displayed on the patient monitor, for example, atrial fibrillation and ST segment depression. This feature allows the operator to alter the ECG tracing on the patient monitor during the course of a simulation indicating a change in patient status. All of this physiological information is stored in the computer log and can be used during the debriefing session to enhance the learning experience for participants.
Programmability of patient simulators
In addition to the features discussed previously, high-fidelity simulators have the capacity to be defibrillated and undergo cardioversion. The physiological response to many drugs and clinical conditions is programmed into some models so that participants can witness the acute response to the administration of medications as well as adverse drug reactions and/or the physiological response to the acute onset of a clinical condition (e.g., acute MI). In addition, instructors can design custom simulations to meet specific instructional needs and learning objectives.
Costs of establishing a simulation laboratory
The costs associated with establishing a simulation laboratory will vary with the type of programmable human patient simulator selected, additional equipment required and/or desired, and the amount of training needed. High-fidelity programmable human patient simulators can cost $100,000 to $200,000 for top-of-the-line models with state-of-the-art features. However, the price of the simulator(s) is only a portion of the setup costs. Establishing a simulation laboratory will also require (1) space designed for the simulation laboratory; (2) audiovisual equipment; (3) networking infrastructure; and (4) training in how to operate the system. The cost for each of these individual components can vary markedly depending on the type of equipment and infrastructure present as well as the level of sophistication desired in terms of audiovisual and networking capacity.
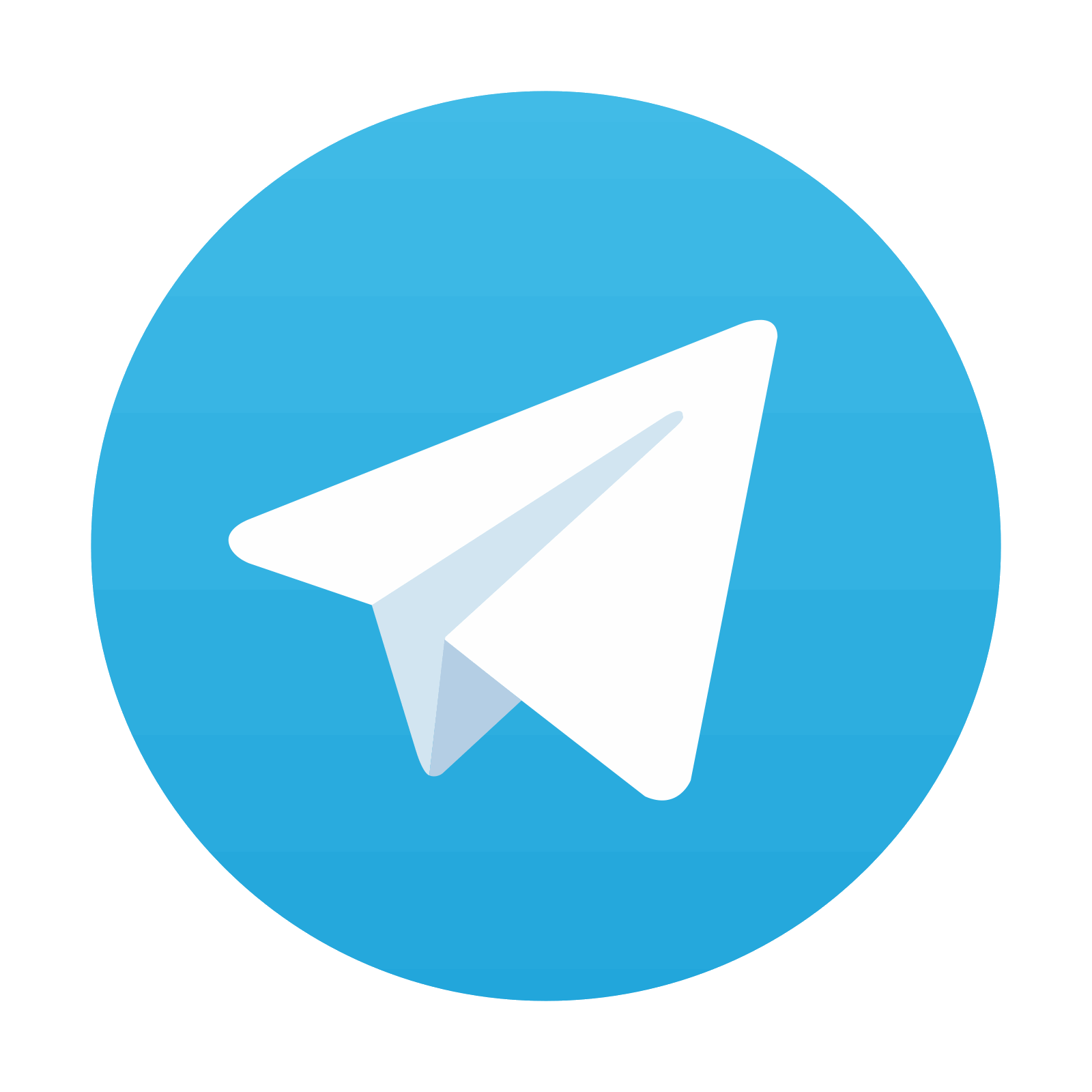
Stay updated, free articles. Join our Telegram channel
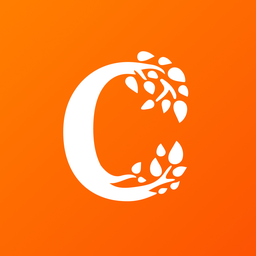
Full access? Get Clinical Tree
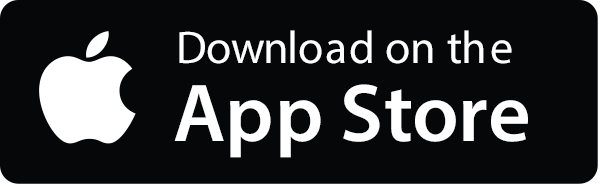
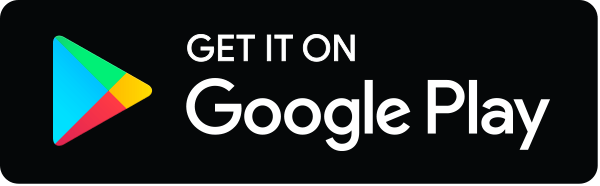