Chapter 4 Early detection of iatrogenic neurologic injury during surgery for spinal deformities is a key step to reducing the incidence and severity of sensorimotor sequelae. After its description in 1973,1 the Stagnara “wake-up” test became the gold standard in many centers for assessing neurologic integrity after the application of corrective forces to a rigid spinal implant. Although this simplified method of assessing motor function has withstood the test of time, it is not without limitations. These include but are not limited to the potential for patient extubation, venous air emboli, false-negative results, poor patient cooperation and cognition during testing. Perhaps the greatest shortcoming of the wake-up test is that it is performed at a single point in time during surgery; namely, after correction.2 This means that spinal cord injury occurring before correction goes undetected until the time of the wake-up test. The temporal delay between the time of insult and that of detection by a wakeup test not only interferes with reliable identification of the specific surgical maneuver responsible for the injury but also delays intervention to facilitate injury reversal. Moreover, clinical manifestation of spinal cord injury does not always present in a tightly time-locked manner to correction of the spinal deformity. When clinical manifestation of injury is delayed, it can be missed by the wake-up test. Given the limitations associated with the wake-up test, intraoperative neurophysiologic monitoring (IONM) of spinal cord function has become standard-of-care in many leading pediatric and adult spine centers. Beginning with the use of somatosensory evoked potentials (SSEPs) almost three decades ago, and including the more recent use of transcranial electric motor evoked potentials (TceMEPs) and electromyography (EMG), IONM now makes it possible to assess the functional integrity of the dorsal sensory and ventral motor spinal cord tracts and nerve roots essentially in real-time, from anesthesia induction to emergence.2,3–6 Despite its overwhelming success in many major university spine centers, however, IONM has not yet achieved the status of “standard-of-care.” This lack of universal acceptance has been attributed to several factors, including but not limited to, cost considerations, the poorly understood role of IONM and its indications by many in the surgical community, and lack of highly qualified and experienced IONM personnel, both on the professional and technical levels. This chapter presents an overview and update of neuromonitoring during surgery for spinal deformities. Topics include descriptions of the anatomical and physiologic basis for each specific neurophysiologic monitoring technique, the pathophysiology of neurophysiologic signal change, and the influence of anesthesia on neurophysiologic responses. SSEPs have been used to monitor scoliosis surgery since the pioneering paper by Nash and co-workers almost three decades ago.7 SSEPs are elicited by stimulating a peripheral nerve; typically, the posterior tibial or peroneal nerve in the lower extremity or the ulnar or median nerve in the upper (Fig. 4–1A). Lower extremity SSEPs permit monitoring of the entire length of the spinal cord, brain stem, and cerebral hemispheres, whereas upper extremity SSEPs monitor only the cervical segment of the spinal cord and cephalad. The ascending sensory volley triggered by posterior tibial or peroneal nerve stimulation enters the spinal cord through dorsal sacral and lumbar nerve roots at several segmental levels (S1-L4) and may ascend the spinal cord via multiple pathways. The general consensus is that the dorsal or posterior column spinal pathways are the primary sites of mediation for the SSEP.8–11 Other pathways such as the dorsal spinocerebellar and anterolateral tracts may also contribute to the early SSEP responses that are used for monitoring spinal cord function.12–14 Figure 4–1 (A) Comparison of cortical somatosensory evoked potentials (SSEPs) to bilateral stimulation of the posterior tibial nerves recorded using a total intravenous anesthetic (TIVA) regimen (upper trace) versus 1.6% desflurane (lower trace). TIVA maintained with constant infusion of propofol (60 g kg 1 min 1), dexmedetomidine (0.2 g kg 1 h 1), and remifentanil (0.6 µg kg 1 min 1). (B) Comparison of transcranial electric motor evoked potentials (TceMEPs) recorded from the same patient as in Fig. 4–1A using TIVA (upper row of traces) versus desflurane (lower row of traces). Upon ascending the spinal cord, the neural signal enters the medullary nuclei in the brain stem. Because there are no synapses in the ascending pathway until the medullary nuclei, SSEPs recorded up to the level of the lower brain stem predominantly reflect the integrity of peripheral nerve fibers and spinal cord white matter. Beyond the medullary nuclei, the neural pathway mediating the SSEP continues as the internal arcuate fiber system, which crosses the brain stem and ascends as the medial lemniscal pathway, projecting to the thalamus. There is another synaptic junction in the thalamus that in turn projects to sensorimotor cortex where additional synaptic interaction may occur. TceMEPs are neuroelectric events recorded from descending motor pathways, including the corticospinal tract (CST), spinal cord interneurons, anterior horn cells, peripheral nerves, and skeletal muscles after transcranial application of a high-voltage electrical stimulus (Fig. 4–1B). Although motor evoked potentials can also be elicited to transcranial magnetic stimulation, the technical challenges associated with this method preclude its routine use in the operating room.15 CST axons project from cortex through the internal capsule to the caudal medulla. Here, the fibers decussate and descend into the lateral and anterior funiculi of the spinal cord. In contrast with ascending spinal cord axons that mediate SSEPs, descending CST axons that mediate TceMEPs enter the spinal cord gray matter where they interact with spinal interneurons. The axons go on to synapse with motor neurons that innervate peripheral muscle. Lateral CST fibers that synapse in the cervical segment of the spinal cord are arranged medially, followed laterally by fibers that synapse in the thoracic, lumbar, and sacral regions, respectively.15,16 TceMEPs mediated by the CST can be recorded from the spinal epidural or subdural space via a catheter-type electrode or from peripheral musculature using needle electrodes. Responses recorded from the epidural space are led by a D-wave triggered by direct depolarization of CST cells. In awake or lightly anesthetized patients, the D-wave is followed by a series of I-waves triggered indirectly via cortical synapses.17 Descending cortical volleys then summate temporally and spatially to excite spinal motor neurons that project to skeletal muscles, triggering compound muscle action potentials. Intraoperative monitoring of D-waves has particular value during excision of intramedullary spinal cord tumors; however, the need to place a recording electrode either percutaneously or through a laminotomy precludes routine use in most spine centers, particularly for cervical surgery. It is both easier and preferable to record myogenic motor responses from upper and lower extremity peripheral muscle for most spine procedures. H-reflexes and F-responses can be recorded intraoperatively to supplement transcranial motor evoked potentials in the rapid detection of acute spinal shock. Both H-reflexes and F-responses aid in the intraoperative assessment of highly integrated spinal cord systems responsible for the control of complex motor behavior. As such, they provide a model for understanding the mechanisms of spinal cord pathophysiology.18,19 Severe acute spinal cord injury can lead to spinal shock that manifests as suppression of H-reflexes and F-responses secondary to hyperpolarization of caudal motor neurons within seconds of the insult.20–22 Despite having many advantages over TceMEPs, including fewer anesthesia-related constraints, H-reflexes and F-responses tend to be highly variable across patients and seem to be recordable, at least in our experience, in only approximately 60 to 70% of pediatric cases and probably less than 40% of adults. Yet, these responses serve as an excellent cross-check and backup to TceMEPs when they can be obtained. Among the most controversial techniques used to monitor spinal cord function during scoliosis surgery is the neurogenic descending evoked potential. This potential is recorded over lower extremity peripheral nerves (e.g., at the popliteal fossa) in response to transosseous (spinous process, lamina) or epidural electrical stimulation of the spinal cord. Initially, it was thought to be mediated by spinal motor tracts.23 Because of its technical simplicity and robustness in the presence of most anesthetic agents, including neuromuscular blockade, the so-called neurogenic “motor” evoked potential (NMEP) became highly popular in IONM for more than a decade. Unfortunately, its early promise as a measure of motor pathway integrity has not been realized. Numerous studies have shown that both the neurogenic descending evoked potential and SSEP are mediated by common spinal cord pathways. That is, the neurogenic response is not a motor evoked potential but rather a descending potential mediated by antidromic activation of afferent somatosensory spinal cord pathways.24–30 Despite the disappointing evidence that the NMEP reflects sensory rather than motor pathway function, its use should not be dismissed entirely. There are rare occasions where it is not possible to record an SSEP, TceMEP, or H-reflex, and yet, epidural stimulation at the rostral thoracic level elicits a descending neurogenic potential that is recordable over the popliteal fossa.30 Because SSEPs are neither sensitive nor specific in identifying injury to individual spinal nerve roots, owing to their multiple-nerve root mediation, spontaneous electromyography (spEMG) is used to detect mechanical trauma to specific spinal nerve roots during decompression, hook or screw insertion, removal of bony fragments, tumor resection, distraction, or traction. Microtrauma to a spinal nerve root results in ion depolarization, and the resultant neural volley triggers contraction of muscle innervated by that specific nerve root. Abrupt traction of a spinal nerve root or mechanical contact by a surgical instrument will elicit intermittent EMG “burst” or sustained EMG “train” activity. Gradual traction may elicit smaller or even no responses. Transpedicular screw fixation has become an accepted adjuvant to corrective spine surgery. Because the pedicle is intimate with adjacent neural element (i.e., nerve root), the margin of error for proper screw placement is small, carrying potential morbidity (e.g., radicular pain, motor weakness) associated with incorrect screw placement.31–33 Stimulus-evoked electromyography (stEMG) is a quick, simple, safe and effective method for detecting pedicle wall violation during and after pedicle screw fixation.34–42 The technique involves applying an electrical stimulus of known intensity to the pedicle screw head or, in the case of poly-axial screws, the hexagonal port or shaft, and recording EMG activity from muscles innervated by nerve root adjacent to the pedicle. If the pedicle wall is fractured, the nerve root will depolarize at a much lower applied current (<7.0 mA) than if the wall is intact, causing muscle contraction that is recorded as an EMG response (Figs. 4–2A and 4–2B). A chronically compressed and/or irritated nerve root might have thickened dura or fewer/smaller functional fibers available for depolarization. Alternatively, it can be demyelinated, have axonal degeneration, or be ischemic. These conditions result in higher depolarization threshold, which could affect the efficacy of stimulated EMG for detection of cortical perfo-ration. Because a chronically compressed root can show a depolarization threshold of 6 mA to direct stimulation rather than the normal 1 to 3 mA, it is important to adjust the screw stimulation intensity criterion for detection of a pedicle breach. This adjustment is determined empirically by having the surgeon directly stimulate the most pathologic root to determine its threshold.4,43 A tangential benefit of neuromonitoring during surgery for spinal deformity is the ability to use SSEPs and/or TceMEPs to identify impending brachial plexopathy or ulnar nerve neuropathy secondary to malpositioning of the patient. Intermittent monitoring of ulnar nerve SSEPs recorded either directly from the brachial plexus (Erb point) or cervical spine is highly effective in identifying emerging brachial plexopathy or ulnar neuropathy2,3–5,44,45(Fig. 4–3). TceMEPs recorded over deltoid, extensor carpi radialis, and first dorsal interosseous muscles can also provide early warning of emerging brachial plexus or peripheral nerve injury. By convention, all evoked potentials are evaluated in terms of measured amplitude (voltage), latency (ms), and morphology (shape). To be accurate, interpretation of any change in these parameters must be considered relative to known or presumed underlying pathophysiologic processes. Otherwise, interpretation will be subjected to false-positive or false-negative error. Figure 4–2 (A) Compound muscle action potentials (CMAPs) recorded during stimulation of a screw in the left L3 pedicle. Stimulation threshold for the left quadriceps muscle innervated by the L3 spinal nerve root is less than 2.6 mA, consistent with a medial breach of the pedicle wall. (B) CMAPs recorded from another patient during stimulation of a screw in the right L2 pedicle. Stimulation threshold for the right adductor longus muscle innervated by the L2 spinal nerve root is greater than 12 mA, consistent with an intact pedicle wall. In spinal surgery for scoliosis correction, neurologic complications usually are secondary to mechanical (e.g., contusion) and/or ischemic insult. Neural injury in these situations always presents as a loss of evoked potential amplitude. Evoked potential latency almost never changes in the absence of an amplitude loss, other than for reasons associated with increased concentration of inhalational or intravenous anesthetic agents, lowering of core body or limb temperature, or perhaps hypercarbia. Hence, the oft-quoted 10% latency prolongation rule commonly used to define a significant SSEP change creates an inordinate number of false-positive alerts. If injury occurs, whether due to contusion or ischemia, a cascade of physiologic change involving sodium, potassium, and calcium channels occurs. This cascade causes blockage of axonal transmission while leading ultimately to an uncoupling of oxidative phosphorylation, thereby precluding ATP production.46,47 The net result is loss of cellular function and structural integrity, which manifests as a drop in evoked potential amplitude, not a prolongation of latency.3,4,7,43
Intraoperative Neuromonitoring in Spinal Deformity Surgery
♦ Neurophysiologic Monitoring Techniques
Somatosensory Evoked Potentials
Transcranial Electric Motor Evoked Potentials
H-Reflex and F-Response
Neurogenic Descending Evoked Potentials
Electromyography
Brachial Plexus Monitoring
♦ Pathophysiology of Evoked Potential and Electromyography Changes
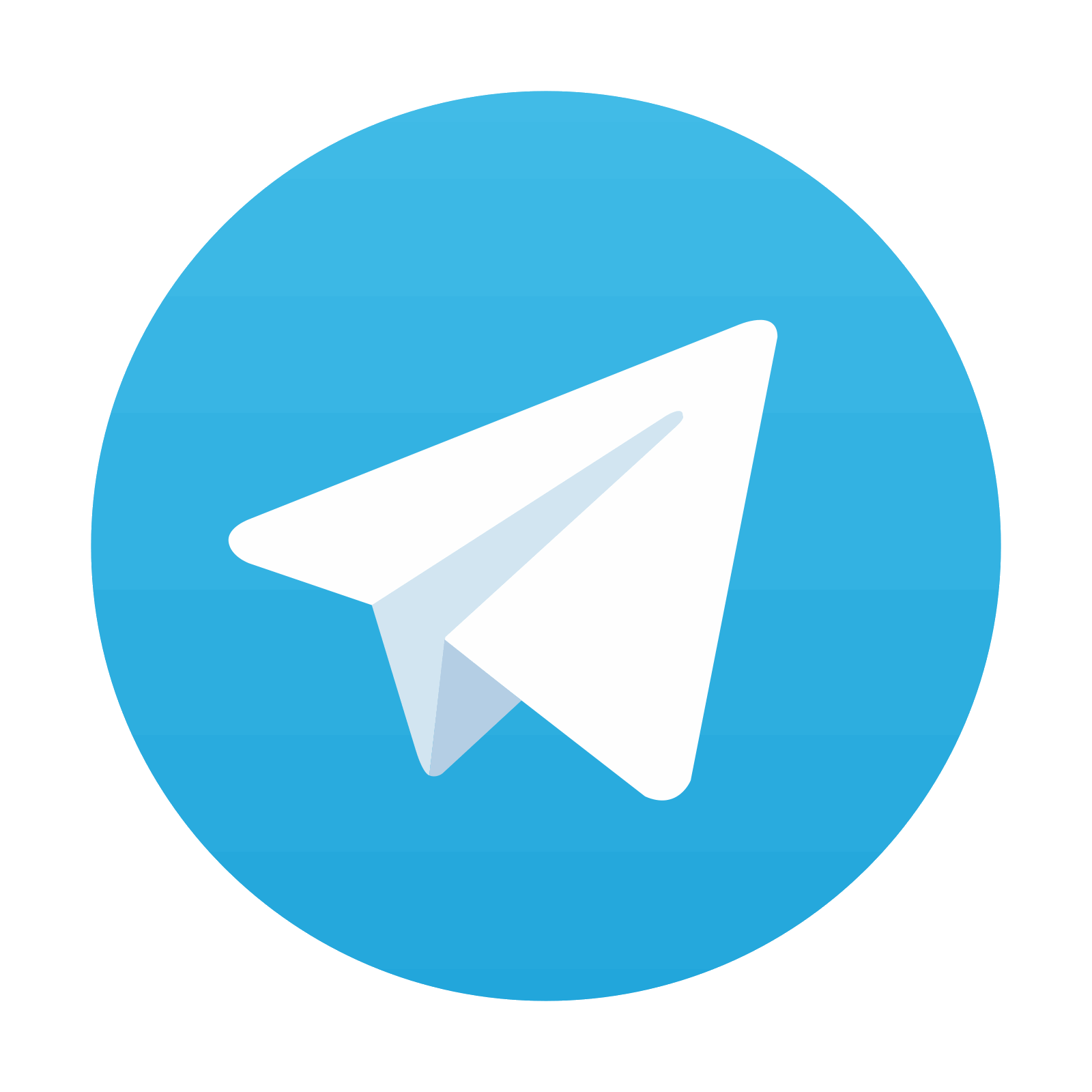
Stay updated, free articles. Join our Telegram channel
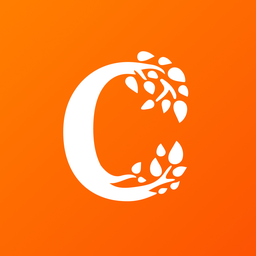
Full access? Get Clinical Tree
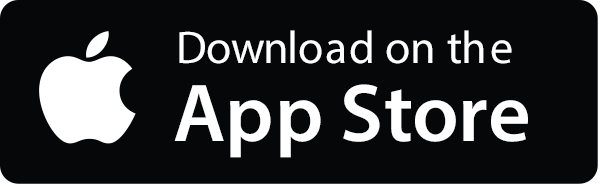
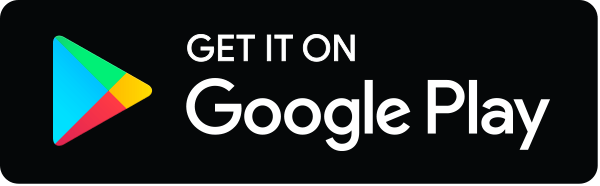