Chapter 31 Introduction
Master Circadian Clock and Master Circadian Rhythm
One of the distinguishing characteristics of sleep in animals as diverse as insects, fish, and mammals is that the timing of sleep and wake for the vast majority of species is rigidly confined to certain times of the day or night. As detailed in a number of chapters in this section, the master biological clock regulating the timing of sleep and wake in mammals also regulates most, if not all, 24-hour (i.e., circadian) behavioral, physiologic, and biochemical rhythms. This master circadian clock is located in the bilaterally paired suprachiasmatic nucleus (SCN) in the anterior hypothalamus (Chapters 32 to Chapter 34). Although it has been discovered that many tissues and organs can generate circadian rhythms in vitro, and therefore independent of the SCN (Chapters 39 and 40), the SCN remains at the top of the hierarchy of the mammalian circadian clock system.
Similarly, one could argue that the sleep–wake cycle represents the master circadian rhythm; the SCN’s control of this rhythm in turn controls the timing and expression of a multitude of downstream rhythms. Although the expression of many 24-hour rhythms may be primarily under the control of the circadian clock in the SCN, many rhythms largely depend on whether the organism is asleep or awake, regardless of the circadian clock time.1 Undoubtedly, the expression of most rhythms at the behavioral, physiologic, and biochemical levels are regulated by the integration of inputs from the circadian clock and the sleep–wake state of the animal. Indeed, it can be argued that the entire temporal organization of an organism represents some combined effect of circadian clock outputs and the sleep–wake state of the organism. Thus, the circadian and sleep control centers have evolved together to ensure that the timing of internal events relative to one another and to the external environment are coordinated in such a fashion to maximize the survival of the species.
Of particular note of the downstream rhythms regulated by the circadian clock is the feeding rhythm. A number of studies have demonstrated that the timing of food intake can regulate the expression of circadian clock genes in many peripheral tissues,2 which in turn control the 24-hour rhythm in the expression of many clock-controlled genes, perhaps up to 10% of all genes expressed in a given tissue.3 The many interactions among the systems regulating circadian, sleep, and energy balance, from molecular to behavioral rhythms, have led to much interest in the importance of these linkages to obesity, diabetes, and cardiometabolic disorders.2
Integration of the Circadian Clock and Sleep–Wake Systems
The first three chapters in this section deal primarily with the circadian clock system, with a focus on the formal properties and rules that govern the generation of circadian rhythms (Chapter 32), the anatomy of the neural clock system in mammals (Chapter 33) and the physiology of the mammalian circadian clock system (Chapter 34). Chapters that review the molecular and genetic bases for the actual circadian clock core machinery, which has been highly conserved at least from insects to mammals, are included in Section 3. Chapters 35 and 37 involve discussions from different vantage points about how the circadian clock system is highly integrated with the sleep–wake regulatory system.
Chapter 36 focuses on the still mysterious pineal gland hormone, melatonin, and its role as perhaps a bridge between the circadian and sleep systems. Although the synthesis and release of melatonin is under tight regulatory control via circadian neural signals from the SCN, melatonin itself can act as a chronobiotic and influence the timing of SCN-controlled rhythms, and it also appears to be a hypnotic that can directly influence the drive to sleep. The complex nature of the interaction between the clock and sleep regulatory systems require more of an overview, and this is provided later.
Chapter 38 focuses on how the circadian clock and sleep loss—together and independently—regulate neurobehavioral performance. The disruption of circadian rhythms in humans during rapid travel across time zones and during shift work has been linked to a variety of mental and physical abnormalities for many years (see Section 9, Occupational Sleep Medicine), but only in the last few years have animal models begun to emerge that are elucidating the extent of the importance of internal circadian synchronization for the health and well-being of the organism as a whole as well as for the molecular events that are disrupted at the level of cells and tissues (Chapters 39 and 40). The final chapter in this Section (Chapter 41) reviews how the clock system underlies disorders related to the timing of the sleep–wake cycle that can lead to a disruption of the timing of internal rhythms with one another, as well as the timing of the sleep–wake cycle and other rhythms with the external environment.
In the now classic two-process model of Borbély and colleagues in Chapter 35, the timing of sleep and wake is a function of a homeostatic process that defines sleep need as depending on the previous amount of sleep and wake (Process S), and the circadian clock (Process C) that modulates the timing and propensity of sleep. However, as noted by Czeisler and colleagues in Chapter 35, the interactions between the circadian pacemaker and sleep homeostat should not be underestimated, and there is great difficulty in separating these two processes functionally. In addition, recent findings in genetics and anatomy (see Section 3 of this volume and Chapter 33) also tend to blur the distinction between the homeostatic and circadian inputs in the regulation of the sleep–wake cycle.
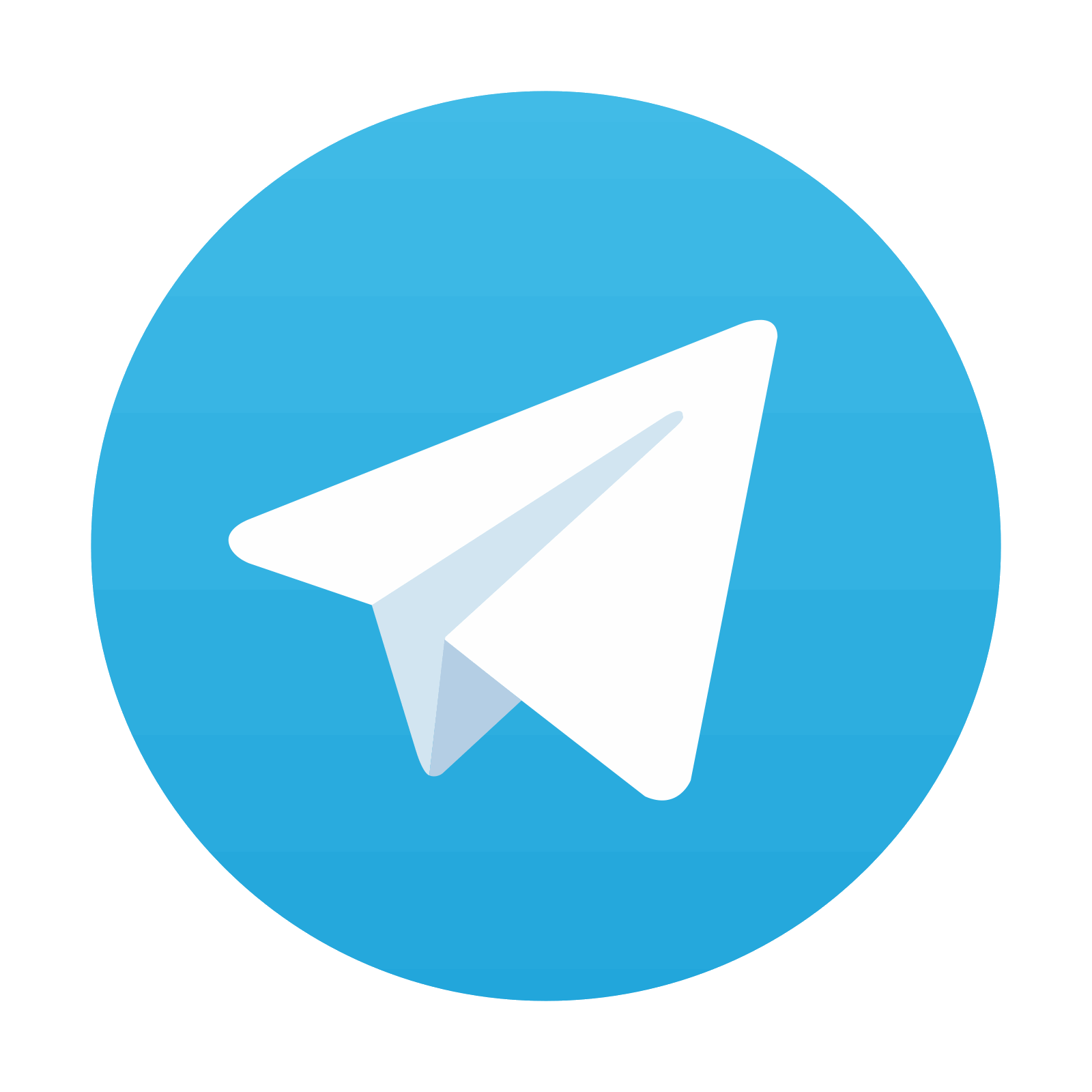
Stay updated, free articles. Join our Telegram channel
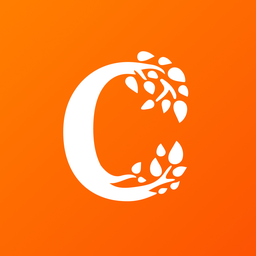
Full access? Get Clinical Tree
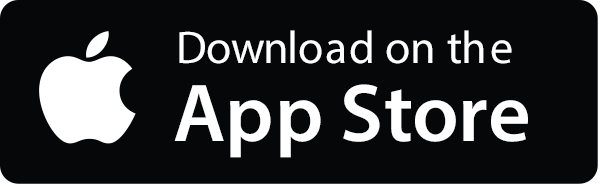
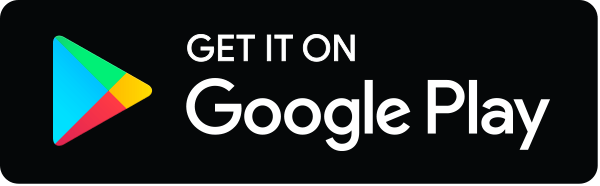