Low-Level Visual Processing: The Retina
The Photoreceptor Layer Samples the Visual Image
Phototransduction Links the Absorption of a Photon to a Change in Membrane Conductance
Excited Rhodopsin Activates a Phosphodiesterase Through the G Protein Transducin
Ganglion Cells Transmit Neural Images to the Brain
The Two Major Types of Ganglion Cells Are ON Cells and OFF Cells
The Output of Ganglion Cells Emphasizes Temporal Changes in Stimuli
Several Ganglion Cell Types Project to the Brain Through Parallel Pathways
A Network of Interneurons Shapes the Retinal Output
The Retina’s Sensitivity Adapts to Changes in Illumination
Light Adaptation Is Apparent in Retinal Processing and Visual Perception
THE RETINA IS THE BRAIN’S WINDOW on the world. All visual experience is based on information processed by this neural circuit in the eye. The retina’s output is conveyed to the brain by just one million optic nerve fibers, and yet almost half of the cerebral cortex is used to process these signals. Visual information lost in the retina—by design or deficiency—can never be recovered. Because retinal processing sets fundamental limits on what can be seen, there is great interest in understanding how the retina functions.
On the surface the vertebrate eye appears to act much like a camera. The pupil forms a variable diaphragm, and the cornea and lens provide the refractive optics that project a small image of the outside world onto the light-sensitive retina lining the back of the eyeball (Figure 26–1). But this is where the analogy ends. The retina is a thin sheet of neurons, a few hundred micrometers thick, composed of five major cell types that are arranged in three cellular layers separated by two synaptic layers (Figure 26–2).
Figure 26-1 The eye projects the visual scene onto the retina’s photoreceptors.
A. Light from an object in the visual field is refracted by the cornea and lens and focused onto the retina.
B. In the foveola, corresponding to the very center of gaze, the proximal neurons of the retina are shifted aside so light has direct access to the photoreceptors.
C. A letter from the eye chart for normal visual acuity is projected onto the densely packed photoreceptors in the fovea. Although less sharply focused than shown here as a result of diffraction by the eye’s optics, the smallest discernible strokes of the letter are approximately one cone diameter in width. (Adapted, with permission, from Curcio and Hendrickson 1982.)
Figure 26-2 The retina comprises five distinct layers of neurons and synapses.
A. A perpendicular section of the human retina seen through the light microscope. Three layers of cell bodies are evident. The outer nuclear layer contains cell bodies of photoreceptors; the inner nuclear layer includes horizontal, bipolar, and amacrine cells; and the ganglion cell layer contains ganglion cells and some displaced amacrine cells. Two layers of fibers and synapses separate these: the outer plexiform layer and the inner plexiform layer. (Reproduced, with permission, from Boycott and Dowling 1969.)
B. Neurons in the retina of the macaque monkey based on Golgi staining. The cellular and synaptic layers are aligned with the image in part A. (M ganglion, magnocellular ganglion cell; P ganglion, parvocellular ganglion cell.) (Reproduced, with permission, from Polyak 1941.)
The photoreceptor cells, in the outermost layer, absorb light and convert it into a neural signal, an essential process known as phototransduction. These signals are passed synaptically to bipolar cells, which in turn connect to retinal ganglion cells in the innermost layer. Retinal ganglion cells are the output neurons of the retina and their axons form the optic nerve. In addition to this vertical pathway from sensory to output neurons, the retinal circuit includes many lateral connections provided by horizontal cells in the outer synaptic layer and amacrine cells in the inner synaptic layer (Figure 26–3).
Figure 26-3 The retinal circuitry.
A. The circuitry for cone signals, highlighting the split into ON and OFF pathways as well as the pathway for lateral inhibition in the outer layer. Red arrows indicate sign-preserving connections through electrical or glutamatergic synapses. Gray arrows represent sign-inverting connections through GABA-ergic, glycinergic, or glutamatergic synapses.
B. Rod signals feed into the cone circuitry through the AII amacrine cell, which serves to split the ON and OFF pathways.
The retinal circuit performs low-level visual processing, the initial stage in the analysis of visual images. It extracts from the raw images in the left and right eyes certain spatial and temporal features and conveys them to higher visual centers. The rules of this processing are very plastic. In particular, the retina must adjust its sensitivity to ever-changing conditions of illumination. This adaptation allows our vision to remain more or less stable despite the vast range of light intensities encountered during the course of each day.
In this chapter we discuss in turn the three important aspects of retinal function: phototransduction, preprocessing, and adaptation. We will illustrate both the neural mechanisms by which they are achieved and their consequences for visual perception.
The Photoreceptor Layer Samples the Visual Image
Ocular Optics Limit the Quality of the Retinal Image
The sharpness of the retinal image is determined by several factors: diffraction at the pupil’s aperture, refractive errors in the cornea and lens, and scattering due to material in the light path. A point in the outside world is generally focused into a small blurred circle on the retina. As in other optical devices this blur is smallest near the optical axis, where the image quality approaches the limit imposed by diffraction at the pupil. Away from the axis the image is degraded significantly owing to aberrations in the cornea and lens. The image may be degraded further by abnormal conditions such as light-scattering cataracts or refractive errors such as myopia.
The area of retina near the optical axis, the fovea, is where vision is sharpest and corresponds to the center of gaze that we direct toward the objects of our attention. The density of photoreceptors, bipolar cells, and ganglion cells is highest at the fovea. The spacing between photoreceptors there is well matched to the size of the optical blur circle, and thus samples the image in an ideal fashion. Light must generally traverse several layers of cells before reaching the photoreceptors, but in the center of the fovea, called the foveola, the other cellular layers are pushed aside to reduce additional blur from light scattering (Figure 26-1B). Finally, the back of the eye is lined by a black pigment epithelium that absorbs light and keeps it from scattering back into the eye.
The retina contains another special site, the optic disc, where the axons of retinal ganglion cells converge and extend through the retina to emerge from the back of the eye as the optic nerve. By necessity this area is devoid of photoreceptors and thus corresponds to a blind spot in the visual field of each eye. Because the disc lies nasal to the fovea of each eye, light coming from a single point never falls on both blind spots simultaneously, and thus normally we are unaware of them. We can experience the blind spot only by using one eye (Figure 26–4). The blind spot demonstrates what blind people experience—not blackness, but simply nothing. This explains why damage to the peripheral retina often goes unnoticed. It is usually through accidents, such as bumping into an unnoticed object, or through clinical testing that a deficit of sight is revealed.
Figure 26-4 The blind spot of the human retina. Locate the blind spot in your left eye by shutting the right eye and fixating the cross with the left eye. Hold the book about 12 inches from your eye and move it slightly nearer or farther until the circle on the left disappears. Now place a pencil vertically on the page and sweep it sideways over the circle. Note the pencil appears unbroken, even though no light can reach your retina from the region of the circle. Next move the pencil lengthwise and observe what happens when its tip enters the circle. (Adapted, with permission, from Hurvich 1981.)
The blind spot is a necessary consequence of the inside-out design of the retina, which has puzzled and amused biologists for generations. The purpose of this organization may be to enable the tight apposition of photoreceptors with the retinal pigment epithelium, which plays an essential role in the turnover of retinal pigment and recycles photoreceptor membranes by phagocytosis.
There Are Two Types of Photoreceptors: Rods and Cones
All photoreceptor cells have a common structure with four functional regions: the outer segment, located at the distal surface of the neural retina; the inner segment, located more proximally; the cell body; and the synaptic terminal (Figure 26–5A).
Figure 26-5 Rod and cone photoreceptors have similar structures.
A. Both rod and cone cells have specialized regions called the outer and inner segments. The outer segment, which is attached to the inner segment by a cilium, contains the light-transducing apparatus. The inner segment holds mitochondria and much of the machinery for protein synthesis.
B. The outer segment consists of a stack of membranous discs that contain the light-absorbing photopigments. In both types of cells these discs are formed by infolding of the plasma membrane. In rods, however, the folds pinch off from the membrane so that the discs are free-floating within the outer segment, whereas in cones the discs remain part of the plasma membrane. (Adapted, with permission, from O’Brien 1982; and Young 1970.)
Most vertebrates have two types of photoreceptors, rods and cones, distinguished by their morphology. A rod has a long, cylindrical outer segment within which the stacks of discs are separated from the plasma membrane, whereas a cone often has a shorter, tapered outer segment, and the discs are continuous with the outer membrane (Figure 26-5B).
Rods and cones also differ in function, most importantly in their sensitivity to light. Rods can signal the absorption of a single photon and are responsible for vision under dim illumination such as moonlight. But as the light level increases toward dawn, the electrical response of rods becomes saturated and the cells cease to respond to variations in intensity. Cones are much less sensitive to light; they make no contribution to night vision, but are solely responsible for vision in daylight. Their response is considerably faster than that of rods. Primates have only one type of rod but three kinds of cone photoreceptors, distinguished by the range of wavelengths to which they respond: the L (long-wave), M (medium-wave), and S (short-wave) cones (Figure 26–6).
Figure 26-6 Sensitivity spectra for the three cones and the rod. At each wavelength the sensitivity is inversely proportional to the intensity of light required to elicit a criterion neural response. Sensitivity varies over a large range and thus is shown on a logarithmic scale. The different classes of photoreceptors are sensitive to broad and overlapping ranges of wavelengths. (Reproduced, with permission, from Schnapf et al. 1988.)
The human retina contains approximately 100 million rods and 6 million cones, but the two cell types are differently distributed. The central fovea contains no rods but is densely packed with small cones. A few millimeters outside the fovea rods greatly outnumber cones. All photoreceptors become larger and more widely spaced toward the periphery of the retina. The S cones make up only 10% of all cones and are absent from the central fovea.
The retinal center of gaze is clearly specialized for daytime vision. The dense packing of cone photoreceptors in the fovea sets the limits of our visual acuity. In fact, the smallest letters we can read on a doctor’s eye chart have strokes whose images are just 1–2 cone diameters wide on the retina, a visual angle of about 1 minute of arc (Figure 26-1C). At night the central fovea is blind owing to the absence of rods. Astronomers know that one must look just to the side of a dim star to see it at all. During nighttime walks in the forest we nonastronomers tend to follow our daytime reflex of looking straight at the source of a suspicious sound. Mysteriously, the object disappears, only to jump back into our peripheral field of view as we avert our gaze.
Phototransduction Links the Absorption of a Photon to a Change in Membrane Conductance
As in many other neurons the membrane potential of a photoreceptor is regulated by the balance of membrane conductances to Na+, and K+ ions, whose transmembrane gradients are maintained by metabolically active pumps (see Chapter 6). In the dark, Na+ ions flow into the photoreceptor through nonselective cation channels that are activated by the second messenger cyclic guanosine 3’-5’ monophosphate (cGMP).
Absorption of a photon by the pigment protein sets in motion a biochemical cascade that ultimately lowers the concentration of cGMP, thus closing the cGMP-gated channels and moving the cell closer to the K+ equilibrium potential. In this way light hyperpolarizes the photoreceptor (Figure 26–7). Here we describe this sequence of events in detail. Most of this knowledge derives from studies of rods, but the mechanism in cones is very similar.
Figure 26-7 (Opposite) Phototransduction.
A. The rod cell responds to light. Rhodopsin molecules in the outer-segment discs absorb photons, which leads to the closure of cGMP-gated channels in the plasma membrane. This channel closure hyperpolarizes the membrane and reduces the rate of release of the neurotransmitter glutamate. (Adapted, with permission, from Alberts 2008.)
B. 1. Cyclic GMP (cyclic guanosine 3’-5’ monophosphate) is produced by a guanylate cyclase (GC) and hydrolyzed by a phosphodiesterase (PDE). In the dark the phosphodiesterase activity is low, the cGMP concentration is high, and the cGMP-gated channels are open, allowing the influx of Na+, and Ca2+. In the light rhodopsin (R) is excited by absorption of a photon, then activates transducin (T), which in turn activates the phosphodiesterase; the cGMP level drops, the membrane channels close, and less Na+, and Ca2+ enter the cell. The transduction enzymes are all located in the internal membrane discs, and the soluble ligand cGMP serves as a messenger to the plasma membrane.
2. Calcium ions have a negative feedback role in the reaction cascade in phototransduction. Stimulation of the network by light leads to the closure of the cGMP-gated channels. This causes a drop in the intracellular concentration of Ca2+. Because Ca2+ modulates the function of at least three components of the cascade—rhodopsin, guanylyl cyclase, and the cGMP-gated channel—the drop in Ca2+ counteracts the excitation caused by light.
C. Voltage response of a primate rod and cone to brief flashes of light of increasing intensity. Higher numbers on the traces indicate greater intensities of illumination (not all traces are labeled). For dim flashes the response amplitude increases linearly with intensity. At high intensities the receptor saturates and remains hyperpolarized steadily for some time after the flash; this leads to the afterimages that we perceive after a bright flash. Note that the response peaks earlier for brighter flashes and that cones respond faster than rods. (Reproduced, with permission, from Schneeweis and Schnapf 1995.)
Light Activates Pigment Molecules in the Photoreceptors
Rhodopsin, the visual pigment in rod cells, has two components. The protein portion, opsin, is embedded in the disc membrane and does not by itself absorb visible light. The light-absorbing moiety, retinal, is a small molecule whose 11-cis isomer is covalently linked to a lysine residue of opsin (Figure 26–8A). Absorption of a photon by retinal causes it to flip from the 11-cis to the all-trans configuration. This reaction is the only light-dependent step in vision.
Figure 26-8 Structure of the visual pigments.
A. Rhodopsin, the visual pigment in rod cells, is the covalent complex of a large protein, opsin, and a small light-absorbing compound, retinal. Opsin has 348 amino acids and a molecular mass of approximately 40,000 daltons. It loops back and forth seven times across the membrane of the rod disc. Retinal is covalently attached to a side chain of lysine 296 in the protein’s seventh membrane-spanning region. Absorption of light by 11-cis retinal causes a rotation around the double bond. As retinal adopts the more stable all-trans configuration, it causes a conformational change in the protein that triggers the subsequent events of visual transduction. (Adapted, with permission, from Nathans and Hogness 1984.)
B. Amino acid sequences of cone and rod pigments. Blue circles denote identical amino acids; black circles denote differences. The three types of cone opsins resemble each other and rhodopsin, suggesting that all four evolved from a common precursor by duplication and divergence. The L and M opsins are most closely related, with 96% identity in their amino acid sequences. They are thought to derive from a gene-duplication event approximately 30 million years ago, after Old World monkeys, which have three pigments, separated from New World monkeys, which generally have only two.
The change in shape of the retinal molecule causes a conformational change in the opsin to an activated state called metarhodopsin II, which triggers the second step of phototransduction. Metarhodopsin II is unstable and splits within minutes, yielding opsin and free all-trans retinal. The all-trans retinal is then transported from rods to pigment epithelial cells, where it is reduced to all-trans retinol (vitamin A), the precursor of 11-cis retinal, which is subsequently transported back to rods.
All-trans retinal is thus a crucial compound in the visual system. Its precursors, such as vitamin A, cannot be synthesized by humans and so must be a regular part of the diet. Deficiencies of vitamin A can lead to night blindness and, if untreated, to deterioration of receptor outer segments and eventually to blindness.
Each type of cone in the human retina produces a variant of the opsin protein. These three cone pigments are distinguished by their absorption spectrum, the dependence on wavelength of the efficiency of light absorption (see Figure 26-6). The spectrum is determined by the protein sequence through the interaction between retinal and certain amino-acid side chains near the binding pocket. Red light excites L cones more than the M cones, whereas green light excites the M cones more. Therefore the relative degree of excitation in these cone types contains information about the spectrum of the light, independent of its intensity. The brain’s comparison of signals from different cone types is the basis for color vision.
In night vision only the rods are active, so all functional photoreceptors have the same absorption spectrum. A green light consequently has exactly the same effect on the visual system as a red light of a greater intensity. Because a single-photoreceptor system cannot distinguish the spectrum of a light from its intensity, “at night all cats are gray.” By comparing the sensitivity of a rod to different wavelengths of light, one obtains the absorption spectrum of rhodopsin. It is a remarkable fact that one can measure this molecular property accurately just by asking human subjects about the appearance of various colored lights (Figure 26–9). The quantitative study of perception, or psychophysics, provides similar insights into other mechanisms of brain processing.
Figure 26-9 Absorption spectrum of rhodopsin.
This plot compares the absorption spectrum of human rhodopsin measured in a cuvette and the spectral sensitivity of human observers to very dim light flashes. The psychophysical data have been corrected for absorption by the ocular media. (Reproduced, with permission, from Wald and Brown 1956.)
Excited Rhodopsin Activates a Phosphodiesterase Through the G Protein Transducin
Activated rhodopsin, in the form of metarhodopsin II, diffuses within the disc membrane where it encounters transducin, a member of the G protein family (Chapter 11). As is the case for other G proteins, the inactive form of transducin binds a molecule of guanosine diphosphate (GDP). Interaction with metarhodopsin II promotes the exchange of GDP for guanosine triphosphate (GTP). This leads to dissociation of transducin’s subunits into an active α subunit carrying the GTP (Tα-GTP) and the β and γ subunits (Tβγ). Metarhodopsin II can activate hundreds of additional transducin molecules, thus significantly amplifying the cell’s response.
The active transducin subunit Tα-GTP forms a complex with a cyclic nucleotide phosphodiesterase, another protein associated with the disc membrane. This interaction greatly increases the rate at which the enzyme hydrolyzes cGMP to 5’-GMP. Each phosphodiesterase molecule can hydrolyze more than 1,000 molecules of cGMP per second, thus increasing the degree of amplification.
The concentration of cGMP controls the activity of the cGMP-gated channels in the plasma membrane of the outer segment. In darkness, when the cGMP concentration is high, a sizeable Na+ influx through the open channels maintains the cell at a depolarized level of approximately –40 mV. As a consequence, the cell’s synaptic terminal continuously releases the transmitter glutamate. The light-evoked decrease in cGMP results in the closure of the cGMP-gated channels, thus reducing the inward flux of Na+ ions and hyperpolarizing the cell (Figure 26–7B1). Hyperpolarization slows the release of neurotransmitter from the photoreceptor terminal, thereby initiating a neural signal.
Multiple Mechanisms Shut Off the Cascade
The photoreceptor’s response to a single photon must be terminated so that the cell can respond to another photon. Metarhodopsin II is inactivated through phosphorylation by a specific rhodopsin kinase followed by binding of the soluble protein arrestin, which blocks the interaction with transducin.
Active transducin (Tα-GTP) has an intrinsic GTPase activity, which eventually converts bound GTP to GDP. Tα-GDP then releases phosphodiesterase and recombines with Tβγ, ready again for excitation by rhodopsin. Once the phosphodiesterase has been inactivated, the cGMP concentration is restored by a guanylate cyclase that produces cGMP from GTP. At this point the membrane channels open, the Na+ current resumes, and the photoreceptor depolarizes back to its dark potential.
In addition to these independent mechanisms that shut off individual elements of the cascade, an important feedback mechanism ensures that large responses are terminated more quickly. This is mediated by a change in the Ca2+ concentration in the cell. Calcium ions enter the cell through the cGMP-gated channels and are extruded by rapid cation exchangers. In the dark the intracellular Ca2+ concentration is high; but during the cell’s light response when the cGMP-gated channels close, the Ca2+ level drops quickly to a few percent of the dark level.
This reduction in Ca2+ concentration modulates the biochemical reactions in many ways (Figure 26–7B2). Rhodopsin phosphorylation is accelerated through the action of the Ca2+-binding protein recoverin on rhodopsin kinase, thus reducing activation of transducin. The activity of guanylyl cyclase is accelerated by Ca2+-dependent guanylyl cyclase-activating proteins. Finally, the affinity of the cGMP-gated channel is increased through the action of Ca2+-calmodulin. All these effects promote the return of the photoreceptor to the dark state.
Defects in Phototransduction Cause Disease
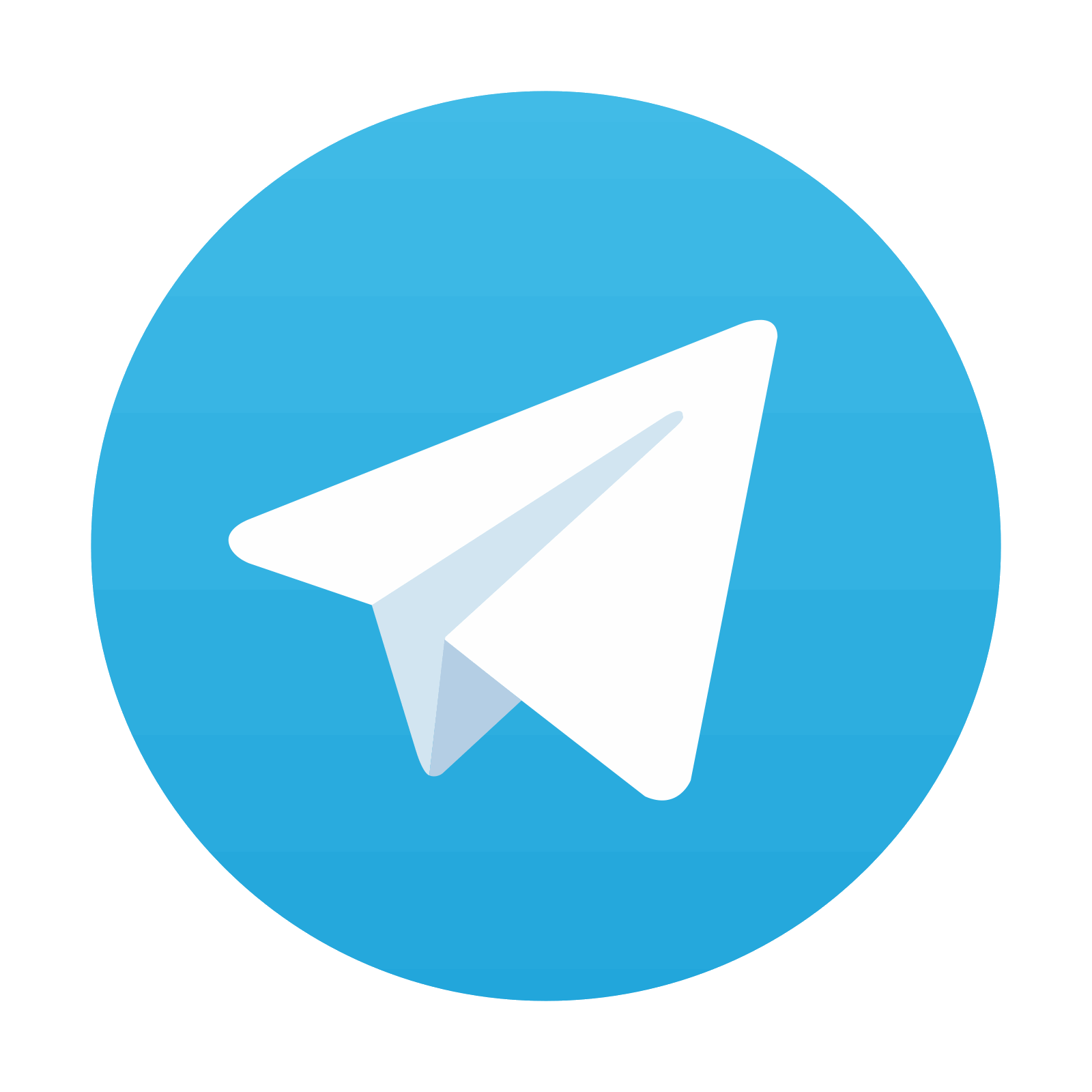
Stay updated, free articles. Join our Telegram channel
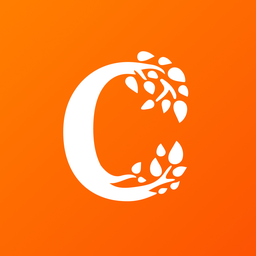
Full access? Get Clinical Tree
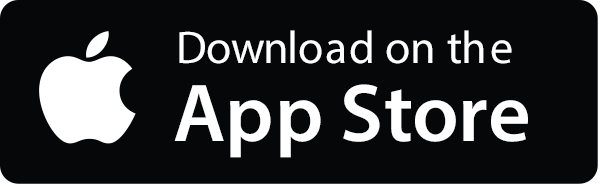
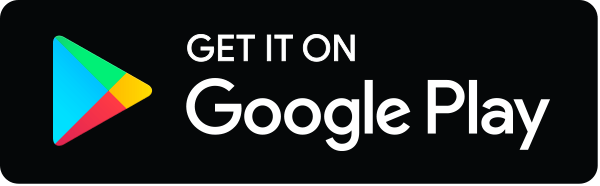